Unravelling the impact of soil types on zinc, iron, and selenium concentrations in grains and straw of wheat/Amblyopyrum muticum and wheat/Triticum urartu doubled haploid lines
- 1School of Biosciences, University of Nottingham, Loughborough, United Kingdom
- 2Department of Sustainable Crops and Soils, Rothamsted Research, Harpenden, United Kingdom
- 3Department of Cop and Soil Sciences, Lilongwe University of Agriculture and Natural Resources, Lilongwe, Malawi
The concentration of mineral nutrients in plants is associated with bioavailabilities of soil mineral nutrients, which are regulated by various soil physio-chemical properties. A pot experiment was conducted to investigate the effects of soil type on grain and straw zinc (Zn), iron (Fe) and selenium (Se) concentrations of wheat/Amblyopyrum muticum and wheat/Triticum urartu doubled haploid lines. A set of 42 treatments in a factorial combination with 21 genotypes and two soil types collected from Ngabu and Chitedze Research Stations in Malawi was laid in a randomised complete block design (RCBD) in three replicates. Pre-experiment soil Zn and Fe were extracted using DTPA extraction method followed by analysis with inductively coupled plasma-mass spectrometry (ICP-MS). Aqua-regia hotplate acid digestion was used to extract soil Se and analysis was done using ICPM-MS. Grain and straw samples were digested using nitric acid digestion (HNO3) and analysed using ICP-MS. Soil analysis results showed that the two soils had the same textural class (Sandy clay loam), but different mineral concentrations, pH levels and percentage organic matter. Analysis of variance revealed a ~two-fold higher Zn concentration in grains grown in low pH, high Zn soils (Chitedze soils) compared to grains grown in high pH, low Zn soils (Ngabu soils). Variation in grain Zn concentration was associated with the genotypes (p = 0002), soil type (p = <0.0001), and their interaction (p = 0.035). Grain Fe was 1.3-fold higher in low pH than in high pH soils, and it was influenced by genotypes (p = < 0.0001) and soil type (p = <0.0001). Grain Se was highly associated with soil type (p = <0.0001), and it was 30-fold higher in high pH than in low pH soils. Straw Zn was generally higher in plants grown in Chitedze soils than Ngabu soils, whilst straw Se was higher in plants grown in Ngabu soils than Chitedze soils. The findings demonstrate the significance of soil physio-chemical properties for mineral accumulation and distribution to plant parts, thus informing future breeding programs on important considerations on crop genetic biofortification with the three mineral elements.
1 Introduction
Micronutrient deficiencies, also called “hidden hunger”, remain a significant public health concern in many countries in sub-Saharan Africa (Phiri et al., 2019; Ligowe et al., 2020; Gashu et al., 2021). Iron (Fe), and zinc (Zn) deficiencies are widespread among women and children, and are among the major causes of anaemia, stunting, cognitive impairment, adverse pregnancy outcomes and increased child mortality and morbidity rates, (Han et al., 2022; Grzeszczak et al., 2020). A study of child stunting from the 2008 to 2020 demographic and health surveys in sub-Saharan Africa (SSA), showed a 35% pooled prevalence of stunting among under-five children (Takele et al., 2022). A 2022 FAO report also revealed that, 40% of all stunted under-five children in 2019 were from Sub-Saharan Africa (FAO, 2022). Unlike stunting, anaemia affects an estimated 40% of children globally, yet in Africa this percentage increases to 60.2%, and 57.1 compared to only 30% women globally (WHO, 2021; Quamme et al., 2022). Globally, Hidden hunger has been associated with 45% of deaths among under five children (WHO, 2021), while in SSA, 3.5 million under-five children die of malnutrition related problems annually (John-Joy Owalade et al., 2022). The world Bank has estimated that a global economic impact of US$3 trillion annually is associated with malnutrition (World bank, 2023), whilst in Africa, the economic burden of malnutrition is between 3 and 16% of GDP annually (Hoddinott, 2016).
Low dietary diversity and heavy reliance on staple cereals with suboptimal essential micronutrients exacerbate micronutrient deficiency risks in SSA (Gashu et al., 2021; Abdu et al., 2022). In Malawi for instance, most households will receive less than 25% of Selenium (Se), less than 50% of Fe and less than 75% of Zn requirement from a typical maize consumption pattern (Gashu et al., 2021). Studies have shown that micronutrients in cereals are concentrated in the aleurone layer and scutellum of the embryo (Meziani et al., 2021; Gaddameedi et al., 2022), and these are mostly removed during the process of milling/flour polishing (Keller and Zingore, 2022), making them unavailable in diets. Some cereals such as wheat also contains anti-nutritional factors such as phytates, which limit the bioavailability of Zn and Fe (Ram et al., 2020). Availability of soil micronutrients and the influence of different edaphic and biological factors such as pH, redox potential, organic matter dynamics, and soil microbiology. also play a major role in enhancing micronutrient concentration in plants (Masunaga and Fong, 2018; Kumar et al., 2020; Giller and Zingore, 2021). In sub-Saharan Africa, there is evidence of widespread but varying micronutrient deficiencies in arable soils, likely affecting grain micronutrient concentration and subsequent low dietary intake (Kihara et al., 2020; Liu et al., 2021). This is true, particularly for Zn, where a number of studies have shown high prevalence of human Zn deficiency in regions with low soil Zn concentrations (Gupta et al., 2020; Belay et al., 2021; Praharaj et al., 2021).
Alleviating “hidden hunger” is goal 2.2 of the 17 UN sustainable development goals (SDGs), and it seeks “to end all forms of malnutrition by 2030”. Food fortification was recommended by the World Health Organisation (WHO) as a long-term strategy to improve dietary micronutrient intake. There is evidence that large scale fortification improves micronutrient status and health outcomes in low- and middle-income countries (Keats et al., 2019). However, fortified foods are mostly unaffordable and difficult to scale up in resource poor countries, unless the costs of fortification are kept low (Horton and Mannar, 2018; Olson et al., 2021). In recent years, scientists have focused on developing cost-effective, and more sustainable strategies of increasing concentration or bioavailability of essential elements in staple crops, such as agronomic and genetic biofortification. These strategies are particularly targeting low- and middle-income countries whose diets are dominated by cereals, with little or no access to fortified foods and animal source micronutrients. Agronomic biofortification has proved successful in enhancing micronutrient concentrations and bioavailability of food crops, including cereals (Cakmak and Kutman, 2018; Zou et al., 2019; Bhardwaj et al., 2022; Yogi et al., 2023). However, within the sub-Saharan Africa context, agronomic biofortification has been hampered by lack of knowledge, access to inputs and additional costs of fertilisers (Valença et al., 2017; Praharaj et al., 2021). In wheat, genetic biofortification using wheat progenitors and wild relatives, which possess significant natural genetic variability compared to cultivated wheat, has been successfully utilized for breeding micronutrient dense wheat varieties, and enabled the release of biofortified wheat cultivars with 8–12 mg kg–1 Zn above a baseline of 25 mg kg–1 (Singh et al., 2017, Velu et al., 2019; Gupta et al., 2022). Compared to the strategies previously discussed, genetic biofortification has been described as a cost-effective and long-term strategy with potential to increase micronutrient dietary intake in resource poor countries. At the University of Nottingham Wheat Research Centre (WRC), a number of doubled haploid (DH)lines carrying different chromosome segments of wheat wild relatives such as Triticum. urartu (AuAu) and Amblyopyrum muticum (TT), were developed in Chinese Spring and Paragon backgrounds for trait discovery. Preliminary mineral analysis of these DH lines resulted in identification of some lines with high micronutrient levels (Zn, Fe and Se) above the wheat parents, potentially contributed by the wild chromosome segments (unpublished data). Before these materials are utilised as a source of genetic variability for improvement of wheat varieties suitable for SSA environments, experiments have to be conducted to evaluate their micronutrient concentration and phenotypic and phenological responses in different soil types, representative of some soils available in some SSA countries. In Malawi and Ethiopia, Gashu et al., 2021, showed that variation in grain Zn, Fe and Se concentrations within crop species arise due to spatial variation in landscape and soil factors such as pH levels, organic matter, and soil available micronutrients. Thus, this paper describes the effects of soil types on grain and straw Zn, Fe and Se concentrations of wheat/Am. muticum and wheat/T. urartu DH lines, and the relationship between grain concentration of the three mineral elements (Zn, Fe and Se), and selected phenotypic and phenological traits.
2 Materials and methods
2.1 Germplasm
Wheat/T. urartu and wheat/Am. muticum DH lines were developed at the WRC at Nottingham University, UK (King et al., 2019; Grewal et al., 2021). Paragon and Chinese Spring (checks) were also sourced from the WRC, while three Malawian checks (Kadzibonga, Kenya nyati and Nduna) were obtained from stocks retained at Lilongwe University of Agriculture and Natural Resources (LUANAR).
2.2 Soil collection, sample preparation and analysis
Topsoil samples (0 – 20 cm) were collected from a field in Ngabu (16° 45′ S and 34° 89′ E), Chikwawa, Malawi, and Chitedze Research Station (13° 98′ S and 33° 65′ E), Lilongwe, Malawi. Approximately 1000 kg of each soil type was collected using hand hoes. The soils were transported to LUANAR in 50 kg sacks. For analysis, samples were collected across the two selected fields using a zigzag sampling pattern. Soil samples collected from different points were mixed in a bucket to form a composite sample. Soil samples were air-dried, crushed with a pestle and mortar before passing them through a 2 mm sieve. 200 grams of each of the composite soil sample was transferred into zip-loc bags, labelled, and shipped to the University of Nottingham for analysis in the laboratory. Soil pH was determined using a Mettler Toledo calibrated pH meter (Scientific Laboratory Supplies, Leicester, United Kingdom) following suspension of 5g of soil sample in 12.5 ml Milli-Q water (18.2 MΩ cm; 1:2.5 m/v) and shaking for 1 hour on an end-over-end shaker. The DTPA extraction method was used to analyse extractable Zn and Fe (Lindsay and Norvell, 1978), followed by analysis using inductively coupled plasma mass spectrometry (ICP-MS; iCAP Q; Thermo Fisher Scientific, Bremen, Germany). Selenium was analysed using aqua-regia hot plate acid digestion and mineral analysis using ICP-MS (Crosland et al., 1995). Soil samples were analysed alongside blanks, WEPAL Calc-ISE 850 (Wepal-Quasimeme, NL-6700 EC Wageningen, Netherlands) certified reference material and a laboratory reference material (Ethiopian soil). Total N was analysed using the Kjeldahl digestion method, and organic matter was determined using the Walkley and Black method (Walkley and Black, 1934).
2.3 Experimental design and trial management
The experiment was conducted in the winter season of 2021 (May-September) at Lilongwe University of Agriculture and Natural Resources (LUANAR -14.18’S 33.76’ E), Lilongwe, Malawi. A set of 42 treatments in a factorial combination of two soil types and 21 genotypes was laid out under screenhouse conditions, in a randomised complete block design (RCBD) with three replicates. The genotypes comprised of 12 wheat/Am. muticum and four wheat/T. urartu DH lines, along with two UK (Chinese spring and Paragon), and three Malawian (Kenya nyati, Nduna and Kadzibonga) checks. Soils were air-dried and sieved before filling 2 litre pots. Distance between pots was 0.2 m, distance between blocks was 1.0 m, while distance between plants from pot to pot ranged from 0.25 to 0.30 m. One seed was sown in each pot, and a 100% germination percentage was attained in plants grown in Ngabu soils, whilst in Chitedze soils germination was at 86%. DH- 339 did not germinate in all three replication, DH-63 and DH-304 germinated in only one of the replications. Re-sowing was done in the ungerminated pots 6 days after the initial sowing. 10 L water-cans were used for irrigation from sowing to maturity at which point irrigation was withdrawn to allow plants to dry. Basal dressing fertiliser 23%N:10%P:5%K +6%S +1%Zn (SuperFert Fertilisers, Harare, Zimbabwe) was applied 14 days after planting at a rate of 200kg/ha, 3 weeks later UREA (46% N) was applied as top dressing at a rate of 100kg/ha. Basal and top dressing were applied according to the Malawi guide to agriculture production (Ministry of Agriculture and Irrigation, 2020) guidelines. The Malawi government recently approved 23N:10P:5K +6S +1Zn) basal fertiliser with 1% Zn due to severe deficiencies (< 2 mg kg-1) of soil Zn across the country (IFDC et al., 2018). Thus, all basal fertiliser blends for selected cereals and legumes in Malawi have 1% Zn. First weeding was done 4 weeks after planting and subsequent weeding as soon as weeds appeared. Insect pests were controlled by applying Profex Super (Profencfos 40% + Cypermenthrin 4% EC –Kewalram Chanrai group).
2.4 Grain and straw micronutrient analysis
Grain and straw samples were collected using standard procedures (Stangoulis and Sison, 2008). Clean and unbroken grain samples were carefully packaged in small brown envelopes after threshing. Straw samples were oven dried (Memmert oven 100-800, Memmert GmbH Co. Kg) at 75 °C for 24 hours. Each sample was then ground using a laboratory mill (Petern LM 3610, Hagersten, Sweden), which was wiped clean before and after adding each sample. After grinding, each sample was packed in small zip lock bags. Both grain and straw sample from the field experiment were shipped to the University of Nottingham. Grain and straw samples were digested using nitric acid digestion (HNO3) described by Khokhar et al. (2018). Briefly, grain samples were soaked overnight (16 hours) in 8mls of nitric acid (>68% PrimarPlus-Trace analysis grade- Fisher Scientific, Loughborough, UK). Samples were digested using a hot block acid digestion system (Anton Paar Gmbh, Graz, Austria). ~0.4 g of each of the grain samples along with certified reference material (wheat flour 1567b-CRM) and laboratory reference material (Paragon wheat-LRM) were digested using a Multicube 48 digestion block (Anton Paar Gmbh, Graz, Austria) described by Gashu et al. (2021). Two operational blanks were added in each run. The digestion block was set at 105˚C for 2h. Samples were diluted with milliQ water (18.2 MΩ cm; Fisher Scientific UK Ltd, Loughborough, UK) up to 50mls. Straw samples were digested in a microwave digestion platform (Khokhar et al., 2018). 0.2 g of each finely ground sample was weighed in pressure-activated venting vessels (56-ml ‘SMART VENT’, Anton Paar) along with three reference materials (CRM-Tom-1573a, BCR-Hay 129 and LRM-Cabbage) and two operational blanks. The samples were digested in a Multiwave PRO microwave with 41-vessel digestion rotor (41HVT56) set at 1,500 W, 10 min heating to 140 °C, 20 min holding at 140 °C, and 15 min cooling to 55 °C. Following digestion, each tube was made up to a final volume of 24ml by adding 16 ml Milli-Q water, then transferred to a 25-ml universal tube (Sarstedt). Both grain and straw samples were further diluted in ICP tubes using a dilution factor of 1:10. Grain and straw multi-element analysis was undertaken using inductively coupled plasma mass spectrometry as described by Gashu et al. (2021) and Khokar et al. (2018). A total of 147-grain samples and 152 straw samples (three replicates for each sample) including blanks and CRMs were analysed. The mineral-specific recovery for grain samples from the field experiment was 90% Zn, 79% Fe, and 95% Se. For straw samples, Zn and Se-specific recovery from CRMs was 92% and 94% respectively. Fe analysis of the straw samples showed higher than normal values, suggesting contamination by soil or during milling, and these have not been reported in this study. The limit of detection (LOD) values for grain Zn, Fe, and Se were 0.8, 0.7 and 0.005 mg kg-1 respectively, and the LOD values for straw Zn and Se were 0.3 and 0.007 mg kg-1 respectively. The LOD values for grain and straw elements were different because of the differences in the CRMs that were used for each.
2.5 Statistical analysis
Two-way analysis of variance (ANOVA) was performed with Genstat regression using Genstat for windows statistical package, version 21 (VSN, 2022). Correlation analyses were performed in RStudio (version 4.1.3), and correlation heatmaps were also created using the same software (RStudio team, 2022). The statistical linear model considered the response Yijk of the ith genotype in the kth replication within the jth soil type expressed as:
Where μ is the grand mean over all genotypes and soil type, βkj is the effect of the kth block/replicate within the jth soil type, τi is the effect of the ith genotype, δj is the effect of the jth soil type, (τδ)ij is the interaction of the ith genotype in the jth soil type, and eijk is the average error. Pairwise comparison and mean separation was done using Tukey honestly significant difference (HSD) test. Correlation analysis was performed using Pearson correlation tests.
3 Results
3.1 Soil characterisation
Table 1 shows the pre-experiment characterisation of the soil samples collected from Chitedze and Ngabu Research Stations, in Malawi. Although both soils were classified as sandy clay loam, analysis results revealed differences in the soil chemical properties. DTPA-extractable Zn was high in Chitedze soils (1.25 mg kg-1) than in Ngabu soils (0.33 mg kg-1). Concentration of Zn in both soils was very low compared to an average soil Zn in unfertilised and uncontaminated soils which ranges from 10-300 mg kg-1 (Noulas et al., 2018). In Chitedze soils, Fe was slightly higher (1.8 mg kg-1) compared to Ngabu soils (1.5 mg kg-1), nonetheless, the values were lower compared to the typical soil Fe which is relatively abundant in many cultivated soils (Gabriel et al., 2021). Selenium was within the ranges of standard soil Se concentration of 0.125-0.3 mg kg-1 (Imran et al., 2023), with a higher concentration in Chitedze soils (0.2 mg kg-1) compared to Ngabu soils (0.1 mg kg-1). Soil Se, however, was consistent with the median soil Zn for Malawi (0.32 mg/kg-1) reported by Gashu et al. (2020).
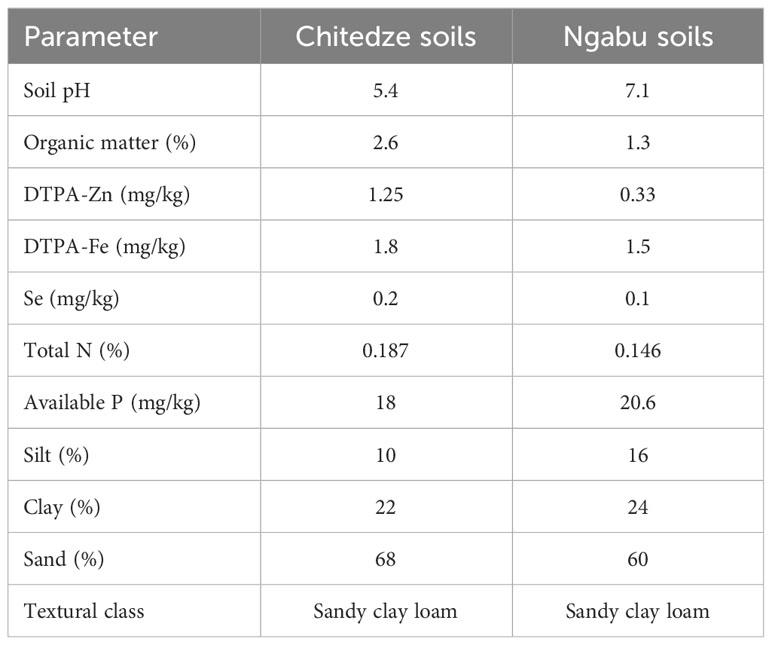
Table 1 Physio-chemical properties of soil samples collected from Ngabu and Chitedze Research Stations.
Soil pH was 5.7 while in Ngabu soils the pH was 7.1. The soils also differed in percentage organic matter, with Chitedze soils having a higher percentage organic matter/carbon than Ngabu soils.
3.2 Grain mineral analysis
3.2.1 Grain Zn concentration
Analysis of grain samples showed a significant variation in grain Zn concentration (Table 2), with 79% of the variation explained by the variables genotypes (p = 0002), soil type (p =<0.0001), and the interaction between soil and genotypes (p = 0.035, F). Among the three variables, mean grain Zn was highly influenced by soil type. Higher grain Zn concentration was observed in soils with lower pH and higher soil Zn (Chitedze) than in higher pH and low soil Zn (Ngabu), and this could be because lower pH values have been associated with high solubility of Zn compared to high pH soils (Neina, 2019, Mossa et al., 2021). In Chitedze soils, mean grain Zn concentration varied from 37.2 to 98.8 mg kg-1 with an overall mean of 69.6 mg kg-1, while in Ngabu soils, mean grain Zn concentration varied from 24.1 to 52.8 mg kg-1 with an overall mean of 39.4 mg kg-1 (Table 2).
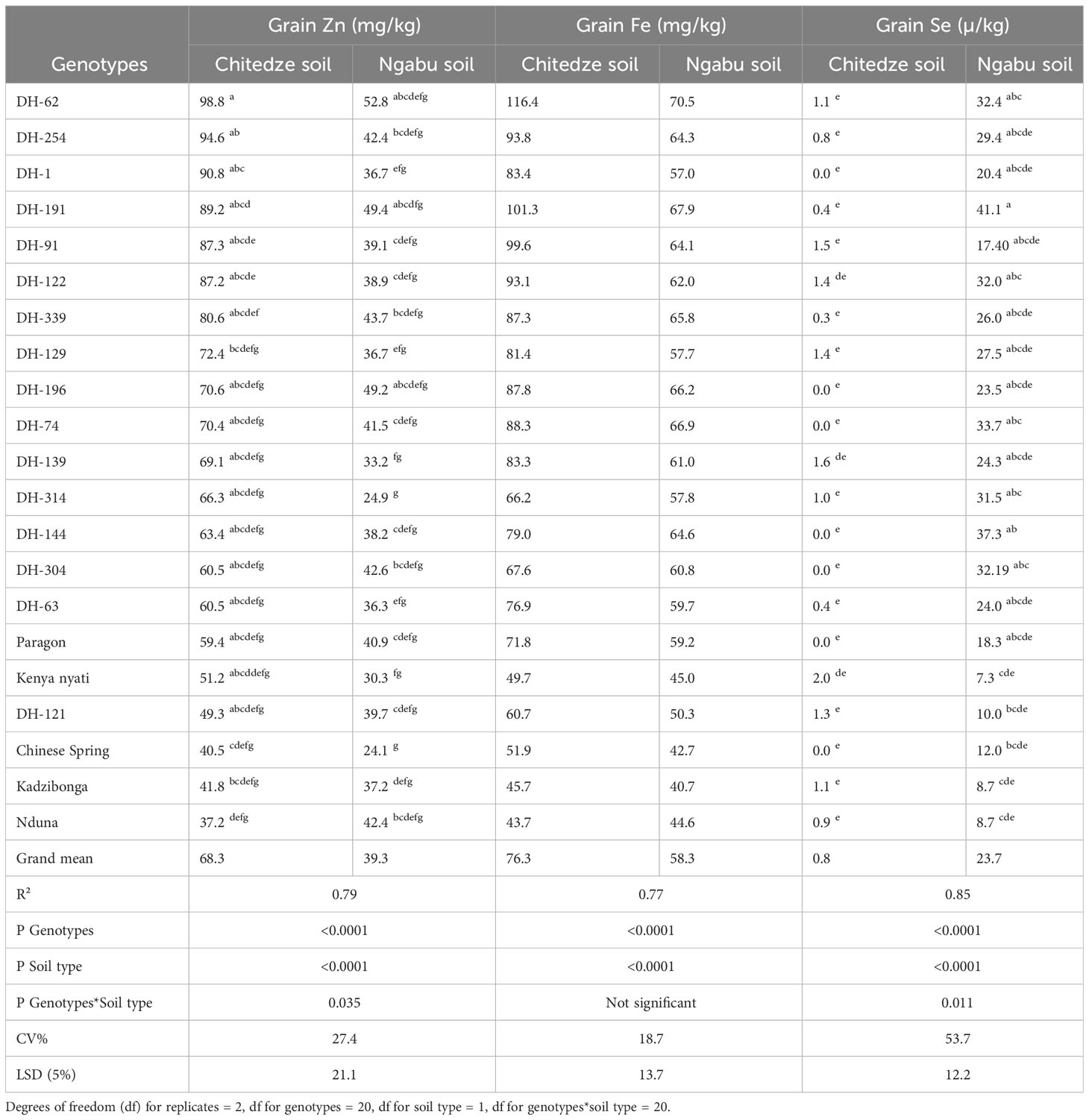
Table 2 Mean variation in grain Zn, Fe and Se concentrations of 21 genotypes grown in Chitedze and Ngabu soils in 2021-winter season. The genotypes have been ordered according to grain Zn concentrations (highest to lowest).
At 95% confidence interval, Tukeys test showed significantly outstanding Zn concentration in DH-62 and DH-254, with up to 30 mg kg-1 Zn higher than the overall mean. Compared to Chinese Spring and Paragon, which are in the background of the DH lines, DH-62 had 58.3 and 39.4 mg kg-1 higher Zn concentration in Chitedze soils respectively, and 74.7 and 57.9 mg kg-1 in Ngabu soils respectively. In both soils, DH-191 also showed significantly higher grain Zn above all the DH lines and the checks, and altogether, these lines could be potential sources of genetic variation for biofortification of locally adaptable varieties. Overall, all the DH lines, with exception of DH-121, showed Zn concentrations above all the five checks in Chitedze soils. In Ngabu soils, five DH lines (62, 191, 196, 339, 304 and 254) showed Zn concentrations above all the five checks.
3.2.2 Grain Fe concentration
Grain Fe concentration was generally higher in wheat grown in Chitedze soils compared to Ngabu soils (Table 2). With an R2 value of 77%, and a P value of<0.0001, soil type and genotypes explained much of the variation, whereas soil type and genotype showed no significant interaction effect (p =< 0.364). The similarity in the trend of grain Zn and Fe in the two soil types informs similar responses to the different physio-chemical propertied of the two elements. Previous studies have shown that QTLs for grain Zn and Fe are co-located (Chandu et al, 2024; Roy et al., 2022), and this could explain the similarity in response. Grain Fe concentration ranged from 43.7-116.4 mg kg -1 in plants grown in Chitedze soils, and 40.7 to 70.5 mg kg-1 in Ngabu soils. Interestingly, grain concentration of all the DH lines in Chitedze soils were above 60 mg kg-1, which is the estimated average requirement for Fe in humans (Velu et al., 2018).
As observed with grain Zn, DH-62, DH-254 and DH-191 also had significantly higher grain Fe above all the DH lines and the checks in both soils. DH-62 and DH-191 are both Am. muticum derivatives with 4T and 7T Am. muticum segments recombined with the 4D and 7A, and 7D wheat chromosomes respectively. DH-254 is a T. urartu derivative with two AuAu segments recombined with chromosome 5A of wheat. Overall, thirteen DH lines showed higher grain Fe concentrations above the check Paragon, whilst three lines had higher Zn concentrations above the three Malawian checks and Chinese Spring, but lower than Paragon.
3.2.3 Grain Se concentration
Analysis of variance showed high significant differences between genotypes, soil types, and significant genotype × soil type interaction effects for grain Se concentration. Among the three variables, grain Se concentration was highly influenced by soil type, which was evident from the high P and F values (P =<0.0001). In high pH soils, grain Se concentration of DH line 191 with a 7T Am. muticum segment showed the highest grain Se with 17.4 µ kg-1 Se concentration over the average Se concentrations of all genotypes at Ngabu, 22-28 µ kg-1 over Chinese Spring and Paragon, and 32 µ kg-1 over the local checks.
All the DH lines, with the exception of DH-121 and DH-91, showed high Se concentrations above the five checks. In Chitedze soils, mean grain Se concentrations were not significantly different (F = 0.586) and varied from zero to 3.2 µ kg-1 with an overall mean of 0.8 µ kg-1. DH-122 was the only line that had a higher Se concentration above Kenya nyati, the Malawian check with the highest Se concentration. Seven of the DH lines along with Paragon and Chinese Spring did not have any detectable Se in the grain.
3.2.4 Straw Zn concentration
Analysis of variance for straw Zn concentration was comparable to that of grain Zn, as it was largely influenced by soil type (p =< 0.0001, F = 90.8). Compared to grain Zn, straw Zn was also higher in wheat grown in Chitedze soils than those in Ngabu soils (Table 3). In Chitedze soils, straw Zn varied from 18.9 to 39.1 mg kg-1 with an overall mean of 27.0 mg kg-1.
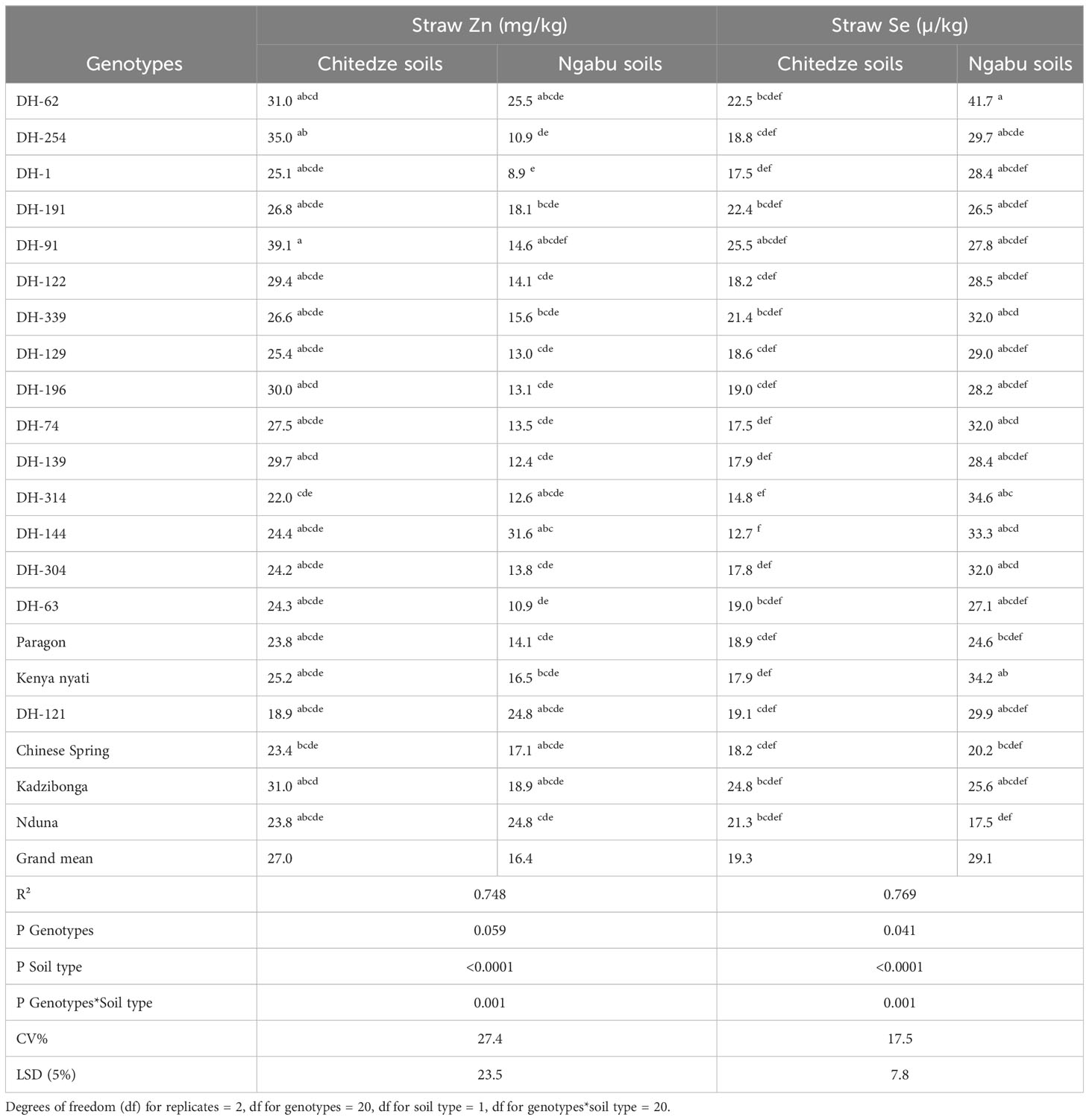
Table 3 Variation in straw Zn and Se of 21 genotypes grown in Chitedze and Ngabu soils in 2021 winter season.
Although DH-91 had the highest straw Zn, DH-254 and DH-62 also had exceptionally higher straw Zn, with over 30 mg kg-1 each. This result could be an indication that the high grain Zn in these two DH lines emanates from the high Zn accumulation in the shoot, and possibly good translocation and remobilisation to the grain. Interestingly, DH-62 also had high straw Zn in Ngabu soils, whereas DH-254 had lower straw Zn. In plants grown in Chitedze soils, Chinese Spring and Paragon had 7.6 and 7.2 mg kg -1 lower straw Zn respectively than DH-62, and 11.6 and 11.2 mg kg-1 lower than DH-254. In the two soil types, straw Zn concentration of the Malawian checks was statistically comparable to, or higher than the straw Zn concentrations of most of the DH lines, although the former had lower grain Zn concentrations. These checks could be better at accumulation of Zn, but possibly have low efficiency to remobilise them to the grain.
3.2.5 Straw Se concentration
Straw Se concentrations varied widely (Table 3), with 75% of the variation explained by the variables genotypes (p = 0.041), soil type (p =<0.0001) and the interaction between soil and genotypes (p = 0.001). Among the three variables, straw Se was highly influenced by soil type, and it was higher in Ngabu soils compared to Chitedze soils. In Ngabu soils, straw Se varied from 17.3 to 41.7 µ kg-1 with an overall mean of 29.1 µ kg-1. DH-62 had the highest straw Se concentration, and it was the only DH line with higher straw Se above the check Kenya nyati. Overall, all the DH lines grown in Ngabu soils showed high straw Se concentration above all the checks except for Kenya nyati. In Chitedze soils, straw Se varied from 12.7 to 25.5 µ kg-1 with an overall mean of 18.8 µ kg-1. DH-91 had significantly higher straw Se concentration and it was the only DH line with higher straw Se above the check Kadzibonga. Three DH lines (191, 62 and 339) also showed higher straw Se concentrations above the check Nduna.
Straw Se concentrations varied widely (Table 3), with 75% of the variation explained by the variables genotypes (p = 0.041), soil type (p =<0.0001) and the interaction between soil and genotypes (p = 0.001). Among the three variables, straw Se was highly influenced by soil type, and it was higher in Ngabu soils compared to Chitedze soils (Figure 1). Mean Straw Se was higher in Ngabu soils compared to Chitedze soils. In Ngabu soils, straw Se varied from 17.3 to 41.7 µ kg-1 with an overall mean of 29.1 µ kg-1. DH-62 had the highest straw Se concentration, and it was the only DH line with higher straw Se above the check Kenya nyati. Overall, all the DH lines grown in Ngabu soils showed high straw Se concentrations above all the checks with the exception of Kenya nyati. In Chitedze soils, straw Se varied from 12.7 to 25.5 µ kg-1 with an overall mean of 18.8 µ kg-1. DH-91 had significantly higher straw Se concentration and was the only DH line with higher straw Se above the check Kadzibonga. Three DH lines (DH-191, DH-62 and DHF1-339) also showed high straw Se concentrations above the check Nduna.
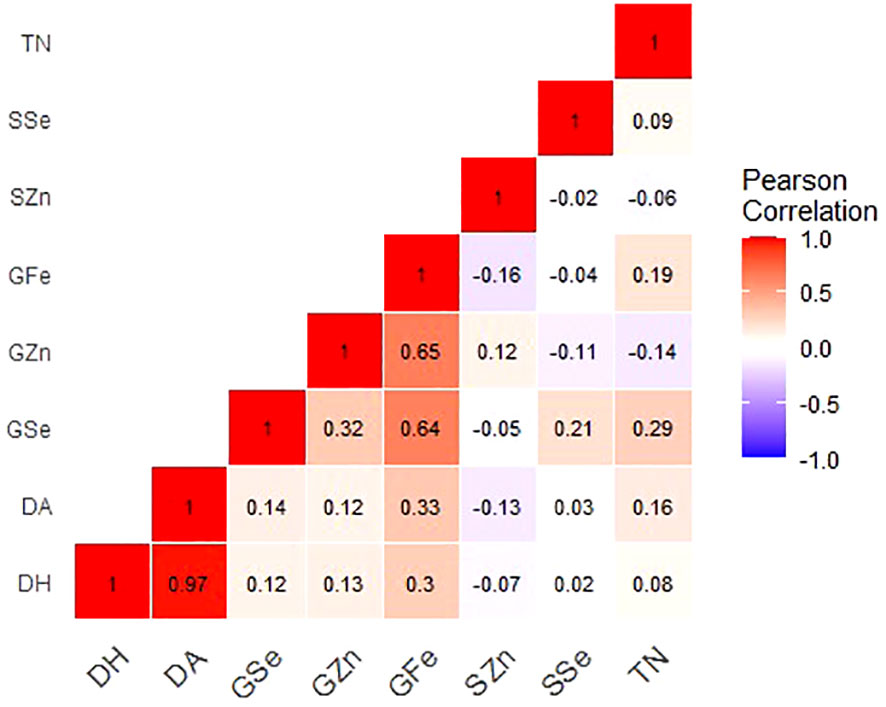
Figure 1 Correlation coefficients for grain mineral-elements and phenotypic and phenological data of Am. muticum and T. urartu DH lines grown in Ngabu soils Note: GZn = grain zinc, GFe = grain iron, GSe = grain selenium, SZn = straw zinc, SSe = straw selenium, TN = number of tillers, DH = days to heading and DA = days to anthesis.
3.2.6 Phenotypic and phenological traits
Significant variation in the number of tillers, days to heading and days to flowering were associated with the variables genotype, soil type and the interaction between genotypes and soil type (Table 4). Although number of tillers per plant varied with genotypes, soil type highly influenced this variability (P =<0.0001, F = 91.3). In Chitedze soils, the number of tillers varied from 2-11 with an overall mean of five, while in Ngabu, number of tillers ranged from one to six with an overall mean of three (see Supplementary Material). This result could be associated with differences in soil available Zn in the two soil types (Table 1). Increased soil Zn concentration through fertilisation has previously been associated with increased number of tillers, grain yield and yield components (Bashir et al., 2023, Hafeez et al., 2021). In this study, although results of weight of grain per plant were collected, yield was not calculated because the DH lines are not adaptable to Malawian conditions, hence their average yield could not be representative.
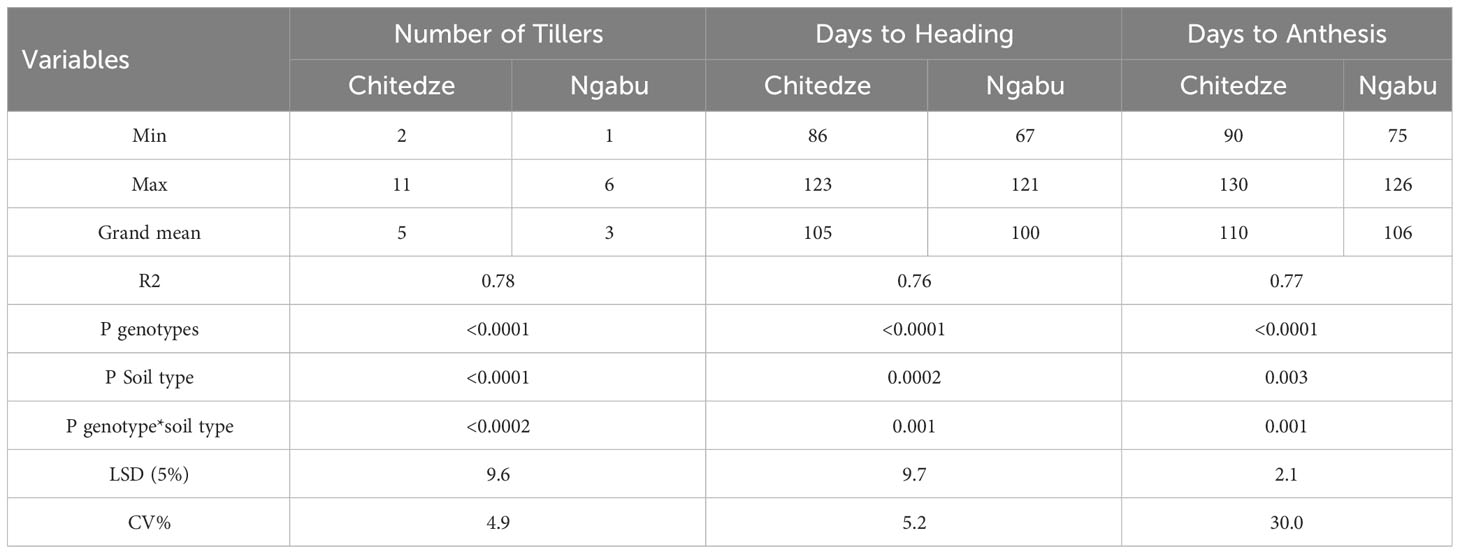
Table 4 Number of tillers, days to flowering and days to heading of 21 genotypes grown in Chitedze and Ngabu soils in the 2021 winter season.
Significant variation in days to heading and anthesis were highly influenced by genotypes compared with soil type and their interaction. In Chitedze soils, days to heading and days to anthesis varied from 86 to 123 and 90-130 respectively, whilst in Ngabu variation was 67 to 121 and 75 to 126 respectively.
3.2.7 Relationship between grain and straw mineral concentrations and agronomic traits
For Chitedze soils (Figure 2), grain Zn concentration showed a significant and very strong positive correlation with grain Fe (r = 0.90, P =<0.001). Grain Zn also positively and significantly correlated with days to anthesis (r = 0.40, P =0.007) and days to heading (r = 0.38, P =0.010). In Chitedze soils, a moderate significant association was observed between straw and grain Zn concentrations (r = 0.41, P = 0.002). Correlation analysis did not show any association between grain and straw Se concentration (r = 0.15, P = 0.106). Grain Fe showed a positive and significant correlation with days to flowering and days to heading, a weak positive correlation with grain Se, and a weak negative correlation with number of tillers. Grain Se showed a weak negative correlation with the three phenotypic traits.
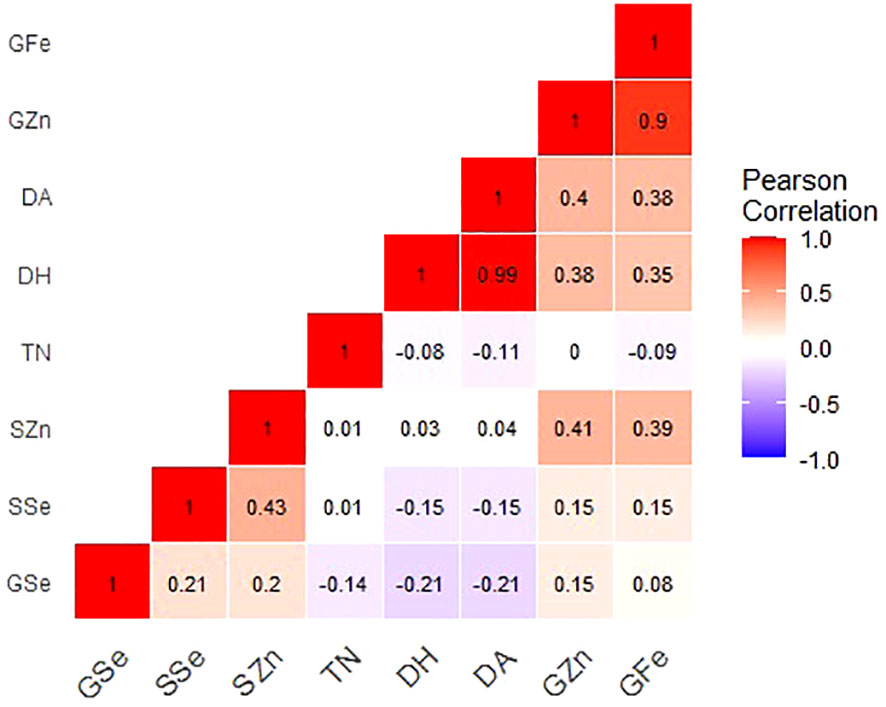
Figure 2 Correlation coefficients for grain mineral-elements and phenotypic and phenological data of Am. muticum and T. urartu DH lines grown in Chitedze soils. Note: GZn = grain zinc, GFe = grain iron, GSe = grain selenium, SZn = straw zinc, SSe = straw selenium, TN = number of tillers, DH = days to heading and DA = days to Anthesis.
In Ngabu soils, grain Zn concentration showed a significant and strong positive correlation with grain Fe (r = 0.650, P =<0.001), and a weak but insignificant positive correlation with grain Se (r = 0.32, P = 0.095). In Ngabu soils, a low positive association was observed between straw and grain Se concentrations (r = 0.21, P = 0.106), while regression analysis did not show any association between grain and straw Zn concentrations (r = 0.12, P = 0.356). Grain Fe significantly and positively correlated grain Se (r = 0.64, P =<0.001), days to anthesis (r = 0.33, P = 0.008), and days to heading (r = 0.31, P = 0.020. Grain Se positively and significantly correlated to number of tillers (r = 0.29, P = 0.023).
4 Discussion
Grain Zn concentrations varied considerably among the 21 genotypes and between the two soil types. Between the soil types, the mean grain Zn concentration for Chitedze soils was ~ two-fold higher than that for Ngabu soils (Table 2). The R2 value from the regression model showed that, variation in grain Zn concentration was highly influenced by soil type, which is likely associated with the differences in soil pH, DTPA-Zn (plant available Zn) and soil organic matter. Soil pH was 7.1 in Ngabu soils while in Chitedze soils pH was 5.4 (Table 1). According to Neina (2019), an increase in soil pH results in the decrease in the solubility of most trace elements, leading to low concentrations in soil solution. Therefore, we assume that the high pH in Ngabu soils resulted in low solubility of soil Zn, making it less available for plant uptake. On the contrary, trace elements are usually soluble in low soil pH, due to high desorption and low adsorption, which could be true for Chitedze soils. Previous work has shown that Zn concentration in soil solution decreases by 30 to 45-fold for each unit increase in soil pH range, specifically from pH 5.5 to 7.0 (Marschner, 1993).
Soil pH is also inversely related to diethylene triamine penta-acetic acid (DTPA)-Zn (Mossa et al., 2021; Dhaliwal et al., 2022), which is also associated with increased grain Zn concentration. For example, post-anthesis Zn accumulation in wheat grains was associated with an increase in DTPA Zn concentration (Liu et al., 2019). A positive correlation between grain Zn and soil DTPA- Zn in wheat, maize and legume growing areas has also been reported (Huang et al., 2019; Manzeke et al., 2019). In this study, DTPA-Zn was 1.25 mg/kg in Chitedze soils while in Ngabu soils DTPA-Zn was 0.33 mg/kg. Barrow and Hartemink (2023) observed that the effects of pH on nutrient availability are not solely caused by the effects on reaction with soils but are an interaction between these effects and the effects on rate of uptake by plants. In winter wheat, Wang et al. (2017) showed that high DTPA-Zn resulted in increased Zn absorption by the root, high translocation of Zn to the shoot with a subsequent increase in the straw. Conversely, low soil DTPA-Zn resulted in low absorption, low translocation to shoot and low straw accumulation (Wang et al., 2017). The findings in the current study suggest that the differences in DTPA-Zn and soil pH between the two soil types, and possibly the rate of plant uptake (as revealed by the significant p value in the genotypes), contributed to the differences in grain Zn concentration. The trend is also reflected in the straw samples, where mean concentrations of straw samples from Chitedze are ~two-fold higher than those from Ngabu soils. Velu et al. (2017) observed that differences in grain Zn concentration between environments were probably caused by different soil Zn levels at each test location (Velu et al., 2018).
Although there is a lot of evidence that accumulation of grain Zn is significantly affected by soil pH and DTPA Zn, the processes are much more complex, considering the synergies that exists between soil Zn and other mineral nutrients. For example, an increase in grain Zn concentration in different cereals was associated with an increase in N application in soils with low available Zn (Jaksomsak et al., 2017, Xue et al., 2019, Khampuang et al., 2021; Hui et al., 2022). These studies have attributed this phenomenon to dilution effect and/or enhanced micronutrient uptake, translocation, remobilization to the grain. On the contrary, an increase in soil P has been associated with a decrease in the concentration of Zn due to the antagonistic interaction between P and Zn, explained by the “dilution effect”, and the suppression of Zn uptake efficiency by roots (Hui et al., 2019; Zhang et al., 2021). Interestingly, growing inherently high Zn cultivars was shown to reduce the P and Zn antagonism by improving Zn activation, acquisition, and translocation at key growth stages (Yang et al., 2023). Studies have shown an improvement in grain Zn and Fe content with a small percentage of Zn and Fe foliar fertilisers (Jalal et al., 2020; Hao et al., 2021; Nanja Reddy et al., 2023), However, soil applied Zn has shown much difference in yield, yield components and other phenotypic traits than in grain Zn concentrations (Nanja Reddy et al., 2023). In this study, 23%N:10%P:5%K +6%S +1%Zn fertiliser was applied as a standard agronomic practice for NPK application in cereals in Malawi. In both soil types, the 1% Zn in the fertiliser could have a positive effect on the soil available Zn and subsequent grain Zn concentration, but just like the N, P and K, the effects of these elements on the accumulation of Zn, Fe and Se were not quantified. Although overall differences in grain Zn concentration could be associated soil physio-chemical properties, sowing dates, particularly for the 3 lines (DH-304, DH-63 and DH-339) that were re-sown 6 days after the initial sowing, could also have an effect on the accumulation of mineral nutrients. In sesame a 16-day difference in sowing resulted in 14 mg kg -1 less grain Zn in the late planted, whilst a sowing difference of 8 days did not show any significant effect on grain Zn and Fe concentration (Bhardwaj et al., 2014). In wheat, a 20-day difference in sowing resulted in high grain Zn concentration in early sown wheat compared to the late sown wheat (Hussain et al., 2023). In this study, a 6-day sowing difference could have a non-significant effect on Zn and Fe grain concentration, however this needs further investigation.
Organic matter can have both a positive and a negative effect on the solubility of Zn for plant uptake. Soils with high rapidly decomposable organic matter result in soluble organic Zn complexes, which are mobile and available for plant uptake (Marschner, 2021), while in high non-decomposable organic matter, Zn may be low due to the formation of stable organic complexes with the solid-state organic matter (Villarino et al., 2023). In this study, organic matter was higher in Chitedze soils compared to Ngabu soils; however, the effect of high organic matter on grain Zn concentration cannot be conclusive. Both Ngabu and Chitedze soils were previously described as vertisols, which fall in the class of calcareous soils often characterised by high pH, high CaCO3 content and low zinc concentration. Soil characterisation in this study shows that the Ngabu soils fit the vertisols description better than the Chitedze soils. Joy et al. (2015) showed that total Zn concentration of leafy vegetables was significantly higher when grown in non-calcareous soils compared to calcareous soils. These findings are similar to their findings.
Variation in grain Zn concentration was also influenced by the genotypes. DH-62 stood out among the genotypes, with the highest grain Zn concentration when grown in both Chitedze and Ngabu soils and high straw Zn for both the soils. This finding suggests high efficiency of DH-62 in Zn uptake, and remobilisation of Zn from straw to grain regardless of soil type. Increased Zn uptake from the soil solution has previously been associated with root type, which is a factor of genotype differences (Liu et al., 2017). A significant positive correlation between grain and straw Zn, particularly in Chitedze soils, supports this argument. Correlation analysis of grain and straw Zn (Figures 1, 2) suggest that some lines were effective in Zn remobilisation to the grain while some were not. Previous studies have shown that root Zn uptake and Zn remobilisation from straw to grain are both essential for grain Zn accumulation (Liu et al., 2019). Wheat/T. urartu DH-254 and wheat/Am. muticum DH-191 also showed exceptionally high grain and straw Zn concentrations in both soil types, although DH-254 showed very low straw concentrations in Ngabu soils. DH-62 is a wheat/Am. muticum DH line with Am. muticum segments on wheat chromosome 4D and 7A. DH-254 has two T. urartu segments recombined with chromosome 5A of wheat, while DH-191 has Am. muticum segments on wheat chromosome 7D. Interestingly, in both the soil types, all the three lines showed high grain Zn concentrations above the checks Paragon and Chinese Spring, which are the wheat background in the DH introgression lines. This result suggests the effect of the wild relative segments on grain Zn accumulation. Similarly, the three DH lines, like most of the DH lines, showed high grain Zn concentrations above the three Malawian wheat checks. This shows that the DH lines are potential sources of novel alleles for biofortification of wheat with Zn.
Variations in grain Fe concentration were also highly influenced by soil type (Table 2). When grown in Chitedze soils, mean grain Fe concentration was 1.3-fold higher than for Ngabu soils. This is more likely because of the differences in soil pH and organic matter. Usually, when soil pH is near or above 7.0, plant available Fe becomes limited due to low solubility (Colombo et al., 2014). Low solubility of Fe is more pronounced in calcareous soils where Fe is rapidly converted into unavailable forms, leading to immobilisation (Ramzani et al., 2016; Ramzani et al., 2017; Tsai and Schmidt, 2020). A previous review has shown that humic substances in organic matter help form stable complexes with metal micronutrients that help maintain micronutrients in bioavailable forms at different pH values (Zanin et al., 2019). In this study, Chitedze soils had higher organic matter compared to Ngabu soils, thus creating more bioavailable Fe. Unlike soil Zn concentrations, soil Fe was not very different between the two soil types; therefore, organic matter levels less likely affected the variation in grain Fe concentrations between the two soil types.
Variation in grain Fe concentrations were also explained by the genotype factor (Table 2). DH-62 and DH-191 had exceptionally high grain Fe concentrations compared to all the genotypes across the soil types. DH-91, DH-254 and DH-122 also had significantly higher grain Fe across both soil types. Interestingly, all these lines also showed significantly higher grain Zn concentrations. Correlation analysis between grain Zn and Fe concentrations revealed a strong positive association, suggesting that the two minerals can be improved simultaneously. This finding is in line with previous studies (Khokhar et al., 2018, 2020). Across the soil types, most of the DH lines had grain Fe concentrations above Paragon and Chinese Spring, and above the three Malawian checks. According to HarvestPlus, to reach 80% of the estimated average requirement of Fe for an adult male, biofortification programs must aim at increasing grain Fe to 60 mg kg-1. In Chitedze and Ngabu soils, 94 and 69% of the DH lines had Fe concentration above 60 mg kg -1, respectively, while all the Malawian checks had less than the EAR, suggesting that the DH lines can be used to improve the Malawian wheats for grain Fe.
Variation in grain Se concentration was highly influenced by soil type, with genotypes grown in Ngabu soils showing a 30-fold higher grain Se than genotypes grown in Chitedze soils (Table 2). Differences in soil chemical properties, particularly soil pH and organic matter likely influenced the availability of Se for uptake and subsequent translocation to the shoot. Se bioavailability is an important factor controlling Se concentration in wheat grains, and generally decreases with decreasing pH, increased organic matter and other soil properties (Stroud et al., 2010; Jones et al., 2017; Liu et al., 2020; Teixeira et al., 2021; Tolu et al., 2022; Xu et al., 2023; Zhangqian et al., 2023). Se uptake also decreases with increased soil organic matter content (Tsioubri et al., 2020). In maize grains, Chilimba et al. (2011) showed a 10-fold higher grain Se concentration in samples grown in calcareous (Eutric vertisols) soils compared with different types of cambisols, luvisols and lixisols. Their study also showed a strong positive correlation between grain Se concentration and soil pH above pH 6·5, which was attributed to decreasing adsorption of inorganic selenate and selenite on iron/manganese oxides (Chilimba et al., 2011). The current study shows that grain Se concentration was high in high pH soils and almost negligible in low pH soils. These findings are also consistent with other previous studies (Xu et al., 2018; Chilimba et al., 2020; Gashu et al., 2020; Ligowe et al., 2020). Effects of soil type on Se concentrations were also reflected in straw samples, with higher straw Se in samples from Ngabu soils compared to Chitedze soils. Correlation analysis showed a significant association between straw and grain Se in Ngabu soils (Figure 1), and a negative association in straw and grain samples grown in Chitedze soils (Figure 2). This could be an indication that the low Se content in the straw samples in Ngabu soils was not remobilised to the grain, while in Chitedze soils, some lines were efficient in remobilisation, while others were not. Although the factor genotypes contributed to the overall variation in grain Se concentration, there was no statistical variation in the genotypes in Chitedze soils. In Ngabu soils, DH-191 and DH-144 showed significantly higher grain Se concentrations than all other genotypes. All the DH lines, with the exception of DH-121 and DH-91, had higher grain Se than all the checks. However, the grain Se concentration in all the genotypes was below the required level (50-100 µg kg -1 of body weight) for adequate intake for humans (NIH, 2021; Avery and Hoffman 2018), therefore making it difficult to select them for improvement of wheat for grain Se.
5 Conclusion and future perspectives
Doubled Haploid lines grown in soils with lower pH (5.4), higher DTPA-Zn (1.25 mg/kg-1) and higher organic matter content (2.6%) showed an overall two-fold higher Zn concentration and 1.3-fold higher Fe than DH lines grown in high pH (7.1), lower DTPA Zn (0.33 mg kg-1) and lower organic matter content (1.3%). Conversely, Se concentrations were 30-fold higher in high pH soils compared to the low pH soils. An indication that differences in soil types (physio-chemical properties) results in substantial variation in grain micronutrient concentrations. The difference in sowing dates of the three DH-lines might have had an effect on the accumulation of the three mineral nutrients and presents a limitation in mineral concentration comparison of the lines in the two soil types. Although Se concentration was higher in the high pH soils, the concentrations were lower than the average requirement for human nutrition, therefore, these lines cannot be very useful for Se biofortification. The majority of the DH lines showed higher grain Zn and Fe concentrations compared to the wheat in their genetic backgrounds, a strong indication of a large genetic diversity in the DH lines for improving the nutritional quality of wheat for enhanced grain Zn and Fe concentration. DH-62, DH-254 and DH-191 will specifically be useful for improving nutritional quality in African adapted genetic backgrounds, with consideration to the different soil types available.
Currently, DH-254 and a sister line to DH-62 have been sequenced, and their T. urartu and Am. muticum introgressions have been successfully transferred to Malawian wheat backgrounds. Preliminary field experiments for the segregating populations are being conducted, and in the future, we plan to identify specific genomic regions underlying the concentration of grain Zn and Fe in the wild chromosomes segments and run a number of trials to test the effects of the chromosome segments in the introgression lines.
Data availability statement
The original contributions presented in the study are included in the article/Supplementary Material. Further inquiries can be directed to the corresponding authors.
Author contributions
VG: Conceptualization, Data curation, Formal analysis, Investigation, Methodology, Software, Writing – original draft, Writing – review & editing. MB: Conceptualization, Funding acquisition, Methodology, Resources, Supervision, Validation, Writing – review & editing. MH: Conceptualization, Methodology, Supervision, Validation, Writing – review & editing. MM: Conceptualization, Supervision, Validation, Writing – review & editing. JB: Conceptualization, Methodology, Supervision, Validation, Writing – review & editing. MB: Data curation, Investigation, Writing – review & editing. SG: Funding acquisition, Resources, Supervision, Writing – review & editing. LW: Formal analysis, Investigation, Methodology, Writing – review & editing. JK: Conceptualization, Funding acquisition, Methodology, Project administration, Resources, Supervision, Validation, Visualization, Writing – review & editing.
Funding
The author(s) declare that financial support was received for the research, authorship, and/or publication of this article. Future Food Beacon of Excellence, University of Nottingham supported Veronicas PhD studentship, including funds for the trial. Mineral analysis of all the samples was funded by the Bill & Melinda Gates Foundation, through the Geo-Nutrition project [INV-009129]. Screenhouse experiment management and seed shipping fees was provided by the University of Nottingham through Research England-Global Challenges Research (GCRF) quality related (QR) funding. Rothamsted Research and the University of Nottingham receives strategic funding from the Biotechnology and Biological Sciences Research Council as part of the Designing Future Wheat [BB/P016855/1] and the Growing Health (BB/X010953/1) Institute Strategic Programmes. Geo-nutrition project also funded the studentship of Veronica at LUANAR. Future Food Beacon Funded the studentship of Veronica at University of Nottingham.
Acknowledgments
The authors would like to acknowledge Dr Liz Bailey, Dr Saul Vazquez Reina and Ken Davis for supporting us through the process of mineral analysis.
Conflict of interest
The authors declare that the research was conducted in the absence of any commercial or financial relationships that could be construed as a potential conflict of interest.
Publisher’s note
All claims expressed in this article are solely those of the authors and do not necessarily represent those of their affiliated organizations, or those of the publisher, the editors and the reviewers. Any product that may be evaluated in this article, or claim that may be made by its manufacturer, is not guaranteed or endorsed by the publisher.
Supplementary material
The Supplementary Material for this article can be found online at: https://www.frontiersin.org/articles/10.3389/fagro.2024.1305034/full#supplementary-material
References
Abdu A. O., Kumssa D. B., Joy E. J. M., Groote H., Lark R. M., Broadley M. R., et al. (2022). Estimates of dietary mineral micronutrient supply from staple cereals in Ethiopia at a District Level. Nutrients 14, 3469. doi: 10.3390/nu14173469
Avery J. C., Hoffmann P. R. (2018). Selenium, selenoproteins, and immunity. Nutrients 10 (9), 1203. doi: 10.3390/nu10091203
Barrow N. J., Hartemink A. E. (2023). The effects of pH on nutrient availability depend on both soils and plants. Plant Soil 487, 21–37. doi: 10.1007/s11104-023-05960-5
Bashir A., Khan Q. U., Alem A., Hendi A. A., Zaman U., Anwar Y., et al. (2023). Zinc and potassium fertilizer synergizes plant nutrient availability and affects growth, yield, and quality of wheat genotypes. Plants 12, 2241. doi: 10.3390/plants12122241
Belay A., Gashu D., Joy E. J. M., Lark R. M., Broadley M. R., Chagumaira C., et al. (2021). Zinc deficiency is highly prevalent and spatially dependent over short distances in Ethiopia. Sci. Rep. 11, 6510. doi: 10.1038/s41598-021-85977-x
Bhardwaj A. K., Chejara S., Malik K., Kumar R., Kumar A., Yadav R. K. (2022). Agronomic biofortification of food crops: An emerging opportunity for global food and nutritional security. Front. Plant Sci. 13, 1055278. doi: 10.3389/fpls.2022.1055278
Bhardwaj H. L., Hamama A. A., Kraemer M., Langham D. R. (2014). Cultivars, planting dates, and row spacing effects on sesame seed yield and mineral composition. J. Agric. Sci. 6, 1. doi: 10.5539/jas.v6n9p1
Cakmak I., Kutman U. B. (2018). Agronomic biofortification of cereals with zinc: a review. Eur. J. Soil Sci. 69, 172–180. doi: 10.1111/ejss.12437
Chandu G., Balakrishnan D., Munnam S. B., Mangrauthia S. K., Sanjeeva Rao D., Neeraja C. N., et al. (2024). Mapping QTLs for grain iron, zinc, and yield traits in advanced backcross inbred lines of Samba mahsuri (BPT5204)/Oryza rufipogon. J. Plant Biochem. Biotechnol. 33, 68–84. doi: 10.1007/s13562-023-00869-7
Chilimba A. D. C., Young S. D., Black C. R., Rogerson K. B., Ander E. L., Watts M. J., et al. (2011). Maize grain and soil surveys reveal suboptimal dietary selenium intake is widespread in Malawi. Sci. Rep. 1, 72. doi: 10.1038/srep00072
Chilimba A. D. C., Young S. D., Broadley M. R. (2020). “The effect of soil type on selenium uptake and recovery by a maize crop,” in 6th International Conference on Selenium in the Environment and Human Health, Shaanxi, Peoples R China, Oct 27-30 2019. 53–54.
Colombo C., Palumbo G., He J. Z., Pinton R., Cesco S. (2014). Review on iron availability in soil: interaction of Fe minerals, plants, and microbes. Soils Sediments 14, 538–548. doi: 10.1007/s11368-013-0814-z
Crosland A. R., Zhao F. J., Mcgrath S. P., Lane P. W. (1995). Comparison of aqua-regia digestion with sodium-carbonate fusion for the determination of total phosphorus in soils by inductively-coupled plasma-atomic emission-spectroscopy (ICP). Commun. Soil Sci. Plant Anal. 26, 1357–1368.
Dhaliwal S. S., Sharma V., Kaur J., Shukla A. K., Hossain A., Abdel-Hafez S. H., et al. (2022). The pedospheric variation of DTPA-Extractable Zn, Fe, Mn, Cu and other physicochemical characteristics in major soil orders in existing land use systems of Punjab, India. Sustainability 14, 29. doi: 10.3390/su14010029
FAO, I UNICEF, WFP AND WHO (2022). “The state of food security and nutrition in the world 2022,” in Repurposing food and agricultural policies to make healthy diets more affordable (FAO., Rome). doi: 10.4060/cc0639en
Gabriel G. V. M., Pitombo L. M., Rosa L. M. T., Navarrete A. A., de Oliveira L. C., Carmo J. B., et al. (2021). The environmental importance of iron speciation in soils: evaluation of classic methodologies. Environ. Monit. Assess. 193, 63. doi: 10.1007/s10661-021-08874-w
Gaddameedi A., Sheraz S., Kumar A., Li K., Shewry P., Gupta R., et al. (2022). The location of iron and zinc in grain of conventional and biofortified lines of sorghum. Cereal Sci. 107, 103531. doi: 10.1016/j.jcs.2022.103531
Gashu D., Lark R. M., Milne A. E., Amede T., Bailey E. H., Chagumaira C., Botoman L., et al. (2020). Spatial prediction of the concentration of selenium (Se) in grain across part of Amhara Region, Ethiopia. Sci. Total Environ. 733, 139231. doi: 10.1016/j.scitotenv.2020.139231
Gashu D., Nalivata P. C., Amede T., Ander L., Broadley M. R., Botoman L., et al. (2021). The nutritional quality of cereals varies geospatially in Ethiopia and Malawi. Nature 594, 71–76. doi: 10.1038/s41586-021-03559-3
Giller K. E., Zingore S. (2021). Mapping micronutrients in grain and soil unearths hidden hunger in Africa. Nature 594, 31–32. doi: 10.1038/d41586-021-01268-5
Grewal S., Guwela V., Newell C., Yang C. Y., Ashling S., Scholefield D., et al. (2021). Generation of doubled haploid wheat-Triticum urartu introgression lines and their characterisation using chromosome-specific KASP markers. Front. Plant Sci. 12. doi: 10.3389/fpls.2021.643636
Grzeszczak K., Kwiatkowski S., Kosik-Bogacka D. (2020). The role of fe, zn, and cu in pregnancy. Biomolecules 10, 1176. doi: 10.3390/biom10081176
Gupta S., Brazier A. K. M., Lowe M. N. (2020). Zinc deficiency in low- and middle-income countries: prevalence and approaches for mitigation. Hum. Nutr. Diet 33, 624–643. doi: 10.1111/jhn.12791
Gupta O. P., Singh A. K., Singh A., Singh G. P., Bansal K. C., Datta S. K. (2022). Wheat biofortification: utilizing natural genetic diversity, genome-wide association mapping, genomic selection, and genome editing technologies. Front. Nutr. 12 (9), 826131. doi: 10.3389/fnut.2022.826131
Hafeez M. B., Ramzan Y., Khan S., Ibrar D., Bashir S., Zahra N., et al. (2021). Application of zinc and iron-based fertilizers improves the growth attributes, productivity, and grain quality of two wheat (Triticum aestivum) cltivars. Front. Nutr. 8, 779595. doi: 10.3389/fnut.2021.779595
Han X., Ding S., Lu J., Li Y. (2022). Global, regional, and national burdens of common micronutrient deficiencies from 1990 to 2019: A secondary trend analysis based on the Global Burden of Disease 2019 study. EClinicalMedicine 44, 101299. doi: 10.1016/j.eclinm.2022.101299
Hao B., Ma J., Jiang L., Wang X., Bai Y., Zhou C., et al. (2021). Effects of foliar application of micronutrients on concentration and bioavailability of zinc and iron in wheat landraces and cultivars. Sci. Rep. 11, 22782. doi: 10.1038/s41598-021-02088-3
Hoddinott J. (2016). The economics of reducing malnutrition in sub-Saharan Africa, Global Panel working paper (London: Global Panel on Agriculture and Food Systems for Nutrition), 21.
Horton S., Mannar V. (2018). Economics of food fortification. in nutrients, dietary supplements, and nutriceuticals. Food Fortification Globalised World 31, 299–304. doi: 10.1016/B978-0-12-802861-2.00031-6
Huang T. M., Huang Q. N., She X., Ma X. L., Huang M., Cao H. B., et al. (2019). Grain zinc concentration and its relation to soil nutrient availability in different wheat cropping regions of China. Soil Tillage Res. 191, 57–65. doi: 10.1016/j.still.2019.03.019
Hui X., Luo L., Wang S., Cao H., Huang M., Shi M., et al. (2019). Critical concentration of available soil phosphorus for grain yield and zinc nutrition of winter wheat in a zinc-deficient calcareous soil. Plant Soil 444, 315–330. doi: 10.1007/s11104-019-04273-w
Hui X., Wang X., Luo L., Wang S., Gao Z., Shi M., et al. (2022). Wheat grain zinc concentration as affected by soil nitrogen and phosphorus availability and root mycorrhizal colonization. Euro. J. Agron. 134, 126469. doi: 10.1016/j.eja.2022.126469
Hussain I., Ijaz M., Ul-Allah S., Sattar A., Nawaz A., Ghaffar A., et al. (2023). Optimum zinc fertilization and sowing date improved growth, yield components, and grain Zn contents of bread wheat under different tillage systems. J. Soil Sci. Plant Nutr. 23, 2344–2353. doi: 10.1007/s42729-023-01185-8
IFDC, AFAP, AGRA (2018) The status of fertilizer recommendation in Malawi: Gaps, challenges and opportunities. Available online at: https://agra.org/wp-content/uploads/2020/08/Malawi-Report_Assessment-of-Fertilizer-Distribution-Systems-and-Opportunities-for-Developing-Fertilizer-Blends.pdf444.
Imran M., Chen Z., Mehmood A., Rukh S., Weixie W., Asghar W., et al. (2023). Distribution of selenium in soils and human health. IntechOpen. doi: 10.5772/intechopen.110636
Jalal A., Shah S., Carvalho , Minhoto , Teixeira , Filho M., llyas M., et al. (2020). Agro-biofortification of zinc and iron in wheat grains. Gesunde Pflanzen 72, 227–236. doi: 10.1007/s10343-020-00505-7
Jaksomsak P., Rerkasem B., Prom-u-thai C. (2017). Responses of grain zinc and nitrogen concentration to nitrogen fertilizer application in rice varieties with high-yielding low-grain zinc and low-yielding high grain zinc concentration. Plant and Soil 411, 101–109. doi: 10.1007/s11104-016-3056-1
John-Joy Owolade A., Abdullateef R. O., Adesola R. O., Olaloye E. D. (2022). Malnutrition: An underlying health condition faced in sub Saharan Africa: Challenges and recommendations. Ann. Med. Surg. (Lond) 82, 104769.
Jones G. D., Droz D., Greve P., Gottschalk P., Poffet D., McGrath S. P., et al. (2017). Selenium deficiency risk predicted to increase under future climate change. Proc. Natl. Acad. Sci. 114, 2848–2853. doi: 10.1073/pnas.1611576114
Joy E. J. M., Broadley M. R., Young S. D., Black C. R., Chilimba A. D. C., Ander E. L., et al. (2015). Responses of grain zinc and nitrogen concentration to nitrogen fertilizer application in rice varieties with high-yielding low-grain zinc and low-yielding high grain zinc concentration. Plant and Soil 411, 101–109. doi: 10.1007/s11104-016-3056-1
Keats E. C., Neufeld L. M., Garrett G. S., Mbuya M. N. N., Bhutta Z. A. (2019). Improved micronutrient status and health outcomes in low- and middle-income countries following large-scale fortification: evidence from a systematic review and meta-analysis. Am. J. Clin. Nutr. 109, 1696–1708. doi: 10.1093/ajcn/nqz023
Keller T., Zingore S. (2022). Influence of hulling, cleaning and brushing/polishing of (Pseudo) cereal grains on compositional characteristics. Foods 12, 2452. doi: 10.3390/foods12132452
Khampuang K., Rerkasem B., Lordkaew S., Chanakan P. (2021). Nitrogen fertilizer increases grain zinc along with yield in high yield rice varieties initially low in grain zinc concentration. Plant Soil 467, 239–252. doi: 10.1007/s11104-021-05090-w
Khokhar J. S., King J., King I. P., Young S. D., Foulkes M. J., De Silva J., et al. (2020). Novel sources of variation in grain zinc (Zn) concentration in bread wheat germplasm derived from Watkins landraces. PloS One 15, e0229107. doi: 10.1371/journal.pone.0229107
Khokar J. S., Sareen S., Tyagi B. S., Singh G., Wilson L., King I. P., et al. (2018). Variation in grain Zn concentration, and the grain ionome, in field-grown Indian wheat. PloS One 13, e0192026. doi: 10.1371/journal.pone.0192026
Kihara J., Bolo P., Kinyua M., Rurinda J., Piikki K. (2020). Micronutrient deficiencies in African soils and the human nutritional nexus: opportunities with staple crops. Environ. Geochem Health 42, 3015–3033. doi: 10.1007/s10653-019-00499-w
King J., Newell C., Grewal S., Hubbart-Edwards S., Yang C. Y., Scholefield D., et al. (2019). Development of stable homozygous wheat/Amblyopyrum muticum (Aegilops mutica) introgression lines and their cytogenetic and molecular characterization. Front. Plant Sci. 10, 34. doi: 10.3389/fpls.2019.00034
Kumar D., Patel K. P., Ramani V. P., Shukla A. K., Meena R. S. (2020). “Management of micronutrients in soil for the utritional security,” in Nutrient dynamics for sustainable crop production. Ed. Meena R. (Springer, Singapore).
Ligowe I. S., Phiri F. P., Ander E. L., Bailey E. H., Chilimba A. D. C., Gashu D., et al. (2020). Selenium deficiency risks in sub-Saharan African food systems and their geospatial linkages. Proc. Nutr. Soc. 79 (4), 457–467. doi: 10.1017/S0029665120006904
Lindsay W. L., Norvell W. A. (1978). Development of a DTPA soil test for zinc, iron, manganese, and copper. Soil Sci. Soc. America J. 42, 421–428. doi: 10.2136/sssaj1978.03615995004200030009x
Liu D. Y., Liu Y. M., Zhang W., Chen X. P., Zou C. Q. (2019). Zinc uptake, translocation, and remobilization in winter wheat as affected by soil application of zn fertilizer. Front. Plant Sci. 10. doi: 10.3389/fpls.2019.00426
Liu N., Wang M., Zhou F., Zhai H., Qi M., Liu Y., et al (2021). Selenium bioavailability in soil-wheat system and its dominant influential factors: A field study in Shaanxi province. China. Sci. Total Environ. 20 (770), 144664. doi: 10.1016/j.scitotenv.2020.144664
Liu D. Y., Zhang W., Pang L. L., Quiang-Zang Y., Wang X., Liu Y-M., et al. (2017). Effects of zinc application rate and zinc distribution relative to root distribution on grain yield and grain Zn concentration in wheat. Plant Soil 411, 167–178. doi: 10.1007/s11104-016-2953-7
Liu S., Yu F., Yang M., Chen Y., Fu Y., Yang J., et al. (2020). Key factors affecting selenite absorption in wheat leaf blades: pH, temperature, light intensity and leaf position. Plant Soil Envion. 66 (9), 431–436. doi: 10.17221/337/2020-PSE
Manzeke M. G., Mtambanengwe F., Watts M. J., Hamilton E. M., Lark R. M., Broadley M. R., et al. (2019). Fertilizer management and soil type influence grain zinc and iron concentration under contrasting smallholder cropping systems in Zimbabwe. Sci. Rep. 9, 6445. doi: 10.1038/s41598-019-42828-0
Marschner H. (1993). “Zinc uptake from soils,” in Zinc in Soils and Plants. Ed. Robson A. D. (kluwer, dordrecht, the Netherlands), 59–77.
Marschner P. (2021). Processes in submerged soils – linking redox potential, soil organic matter turnover and plants to nutrient cycling. Plant Soil 464, 1–12. doi: 10.1007/s11104-021-05040-6
Masunaga T., Fong J. D. (2018). Strategies for increasing micronutrient availability in soil for plant uptake. Mol. Genomic Perspect. Crop Plants 2018, 195–208. doi: 10.1016/B978-0-12-812104-7.00013-7
Meziani S., Nadaud I., Tasleem-Tahir A., Benguella R., Branlard G., et al. (2021). Wheat aleurone layer: A site enriched with nutrients and bioactive molecules with potential nutritional opportunities for breeding. Cereal Sci. 100, 103225. doi: 10.1016/j.jcs.2021.103225
Ministry of Agriculture and Irrigation (MoAI). (2020). Guide to agriculture production and natural resources management in Malawi. Agriculture Communications Branch, Lilongwe, Malawi. 112–124. ISBN 99908-34-00-9
Mossa A.-W., Gashu D., Broadley M. R., Dunham S. J., McGrath S. P., Bailey E. H., et al. (2021). The effect of soil properties on zinc lability and solubility in soils of Ethiopia – an isotopic dilution study. Soil 7, 255–268. doi: 10.5194/soil-7-255-2021
Nanja Reddy Y. A., Narayana Reddy A. B., Nirmalakumari A., Gowda M. V. C., et al. (2023). Foliar application of zinc enhances the grain zinc concentration, whereas the soil application improves the grain yield of finger millet (Eleusine coracana L.). Plant Physiol. Rep. 28, 513–520. doi: 10.1007/s40502-023-00747-3
Neina D. (2019). The role of soil pH in plant nutrition and soil remediation. Appl. Environ. Soil Sci. 2019, 5794869. doi: 10.1155/2019/5794869
NIH (2021). Selenium fact sheet for health professionals. Selenium - Health Professional Fact Sheet (nih.gov).
Noulas C., Tziouvalekas M., Karyotis T. (2018). Zinc in soils, water and food crops. J. Trace Elem Med. Biol. 49, 252–260. doi: 10.1016/j.jtemb.2018.02.009
Olson R., Gavin-Smith B., Ferraboschi C., Kraemer K. (2021). Food fortification: The advantages, disadvantages and lessons from sight and life programs. Nutrients 13, 1118. doi: 10.3390/nu13041118
Phiri F. P., Ander E. L., Bailey E. H., Chilima B., Chilimba A. D. C., Gondwe J., et al. (2019). The risk of selenium deficiency in Malawi is large and varies over multiple spatial scales. Sci. Rep. 9, 6566. doi: 10.1038/s41598-019-43013-z
Praharaj S., Skalicky M., Maitra S., Bhadra P., Shankar T., Brestic M., et al. (2021). Zinc biofortification in food crops could alleviate the zinc malnutrition in human health. Molecules 26, 3509. doi: 10.3390/molecules26123509
Quamme S. H., Iversen P. O. (2022). Prevalence of child stunting in Sub-Saharan Africa and its risk factors. Clin. Nutr. Open Sci. 42, 49–61. doi: 10.1016/j.nutos.2022.01.009
Ram S. N., Narwal S., Gupta O. P., Pandey V., Singh G. (2020). Anti-nutritional factors and bioavailability: approaches, challenges, and opportunities. Food Science Technol. Nutr. 2020, 101–128. doi: 10.1016/B978-0-12-818444-8.00004-3
Ramzani P. M. A., Khalid M., Anjum S., Khan W.-U.-D., Ali S., Hannan F., et al. (2017). Cost-effective enhanced iron bioavailability in rice grain grown on calcareous soil by sulfur mediation and its effect on heavy metals mineralization. Environ. Sci. pollut. Res. 24, 1219–1228. doi: 10.1007/s11356-016-7892-6
Ramzani P. M. A., Khalid M., Naveed M., Ahmad R., Shahid M. (2016). Iron biofortification of wheat grains through integrated use of organic and chemical fertilizers in pH affected calcareous soil. Plant Physiol. Biochem. 104, 284–293. doi: 10.1016/j.plaphy.2016.04.053
Roy C., Kumar S., Ranjan R. D., Kumhar S. R., Govindan V. (2022). Genomic approaches for improving grain zinc and iron content in wheat. Front. Genet. 13, 1045955. doi: 10.3389/fgene.2022.1045955
RStudio Team (2022). RStudio: Integrated Development for R. RStudio (Boston, MA: PBC). Available at: http://www.rstudio.com/.
Singh R., Velu G., Andersson M. S., Bouis H., Jamora N. (2017). Zinc-biofortified wheat: harnessing genetic diversity for improved nutritional quality. Global Crop Diversity Trust Briefs. doi: 10.22004/ag.econ.283982
Stangoulis J. C. R., Sison C. (2008). Crop sampling protocols for micronutrient analysis. Harvestplus. Technical Moograph Series 7. IFPRI, Wachngton, DC, USA.
Stroud J. L., Broadley M. R., Foot I., Fairweather-Tait S. J., Hart D. J., Hurst R., et al. (2010). Soil factors affecting selenium concentration in wheat grain and the fate and speciation of Se fertilisers applied to soil. Plant Soil 332, 19–30. doi: 10.1007/s11104-009-0229-1
Takele B. A., Gezie L. D., Alamneh T. S. (2022). Pooled prevalence of stunting and associated factors among children aged 6-59 months in Sub-Saharan Africa countries: A Bayesian multilevel approach. PloS One 17(10). doi: 10.1371/journal.pone.0275889
Teixeira L. S., Pimenta T. M., Brito F. A. L., Malheiros R. S. P., Arruda R. S., Ribeiro D., et al. (2021). Selenium uptake and grain nutritional quality are affected by nitrogen fertilization in rice (Oryza sativa L.). Plant Cell Rep. 40, 871–880. doi: 10.1007/s00299-021-02685-6
Tolu J., Bouchet S., Helfenstein J., Hausheer O., Winkel L. H. E., Frossard E., et al. (2022). Understanding soil selenium accumulation and bioavailability through size resolved and elemental characterization of soil extracts. Nat. Commun. 13, 6974. doi: 10.1038/s41467-022-34731-6
Tsai H.-H., Schmidt W. (2020). pH-dependent transcriptional profile changes in iron-deficient Arabidopsis roots. BMC Genomics 21, 694. doi: 10.1186/s12864-020-07116-6
Tsioubri M., Gasparatos D., Economou-Eliopoulos M. (2020). Selenium uptake by Lettuce (Lactuca sativa L.) and Berseem (Trifolium alexandrinum L.) as affected by the application of sodium selenate, soil acidity and organic matter content. Plants 9, 605. doi: 10.3390/plants9050605
Valença A. W., Bake A., Brouwer I. D., Giller K. E. (2017). Agronomic biofortification of crops to fight hidden hunger in sub-Saharan Africa. Global Food Secur. 12, 8–14. doi: 10.1016/j.gfs.2016.12.001
Velu G., Crespo Herrera L., Guzman C., Huerta J., Payne T., Singh R. P. (2019). Assessing genetic diversity to breed competitive biofortified wheat with enhanced grain Zn and Fe concentrations. Front. Plant Sci. 9, 1971. doi: 10.3389/fpls.2018.01971
Velu G., Singh R. P., Crespo-Herrera L., Drasigacker S., Valluru R., Stagoulis J., et al. (2018). Genetic dissection of grain zinc concentration in spring wheat for mainstreaming biofortification in CIMMYT wheat breeding. Sci. Rep. 8, 13526. doi: 10.1038/s41598-018-31951-z
Velu G., Tutus Y., Gomez-Beccera H. F., Hao Y., Cakmak I. (2017). QTL mapping for grain zinc and iron concentrations and zinc efficiency in a tetraploid and hexaploid wheat mapping populations. Plant Soil 411, 81–99. doi: 10.1007/s11104-016-3025-8
Villarino S. H., Talab E., Contisciani L., Videla C., Geronimo P. D., Jackson R. B., et al. (2023). A large nitrogen supply from the stable mineral-associated soil organic matter fraction. Biol. Fertil Soils 59, 833–841. doi: 10.1007/s00374-023-01755-z
Walkley A., Black I. A. (1934). An examination of the degtjareff method for determining soil organic matter, and a proposed modification of the chromic acid titration method. Soil Sci. 37, 29–38. doi: 10.1097/00010694-193401000-00003
Wang S., Wang Z., Gao Y., Liu L., Yu R., Jin J., et al. (2017). EDTA alone enhanced soil zinc availability and winter wheat grain Zn concentration on calcareous soil. Environ. Exp. Bot. 141, 19–27. doi: 10.1016/j.envexpbot.2017.06.008
WHO (2021) Malnutrition. Available online at: https://www.who.int/en/news-room/fact-sheets/detail/malnutrition (Accessed 26 August 2021).
World Bank (2023) The World Bank and nutrition. Available online at: https://www.worldbank.org/en/topic/nutrition/overview.
Xu Y., Li Y., Li H., Wang L., Liao X., Wang J., et al. (2018). Effects of topography and soil properties on soil selenium distribution and bioavailability (phosphate extraction): A case study in Yongjia County, China. Sci. Total Environ. 633, 240–248. doi: 10.1016/j.scitotenv.2018.03.190
Xu Z., Zhou W., Zhou Y., Cui H., Liu R., Shang G. (2023). Factors controlling accumulation and bioavailability of selenium in paddy soils: A case study inuxi County, China. Environ. pollut. 123196. doi: 10.1016/j.envpol.2023.123196
Xue Y. F., Yue S. C., Liu D. Y., Zhang W., Chen X., Zou C. (2019). Dynamic zinc accumulation and contributions of pre-and/or post-silking zinc uptake to grain zinc of maize as affected by nitrogen supply. Front. Plant Sci. 10, 1203. doi: 10.3389/fpls.2019.01203
Yang J., Xu J., Wang Z., Zhang X., Guo Z., Wang L., et al. (2023). High-Zn wheat alleviates P-Zn antagonism by improving Zn activation, acquisition, and translocation at key growth stages. Field Crops Res. 304, 109149. doi: 10.1016/j.fcr.2023.109149
Yogi A. K., Bana R. S., Bamboriya S. D., Choundhary R., Chaundhary A., Singh D., et al. (2023). Foliar zinc fertilization improves yield, biofortification and nutrient-use efficiency of upland rice. Nutr. Cycl Agroecosyst 125, 453–469. doi: 10.1007/s10705-023-10270-4
Zanin L., Tomasi N., Cesco S., Varanini Z., Pinton R. (2019). Humic substances contribute to plant iron nutrition acting as chelators and biostimulants. Front. Plant Sci. 10. doi: 10.3389/fpls.2019.00675
Zhang W., Zhang W., Wang X., Liu D., Zou C., Chen X., et al. (2021). Quantitative evaluation of the grain zinc in cereal crops caused by phosphorus fertilization. A meta-analysis. Agron. Sustain. Dev. 41, 6. doi: 10.1007/s13593-020-00661-0
Zhangqian Xu, Zhou W., Zhou Y., Liu R., Shang G. (2023). Factors controlling accumulation and bioavailability of selenium in paddy soils: A case study in Luxi County, China. Environ. pollut. 23, 123196. doi: 10.1016/j.envpol.2023.123196
Keywords: wheat, micronutrients, biofortification, wild relatives, soil pH, organic matter
Citation: Guwela VF, Broadley MR, Hawkesford MJ, Maliro MFA, Bokosi J, Banda M, Grewal S, Wilson L and King J (2024) Unravelling the impact of soil types on zinc, iron, and selenium concentrations in grains and straw of wheat/Amblyopyrum muticum and wheat/Triticum urartu doubled haploid lines. Front. Agron. 6:1305034. doi: 10.3389/fagro.2024.1305034
Received: 30 September 2023; Accepted: 22 February 2024;
Published: 08 March 2024.
Edited by:
Marloes van Loon, Wageningen University and Research, NetherlandsReviewed by:
Shahid Hussain, Bahauddin Zakariya University, PakistanArshad Jalal, King Abdullah University of Science and Technology, Saudi Arabia
Copyright © 2024 Guwela, Broadley, Hawkesford, Maliro, Bokosi, Banda, Grewal, Wilson and King. This is an open-access article distributed under the terms of the Creative Commons Attribution License (CC BY). The use, distribution or reproduction in other forums is permitted, provided the original author(s) and the copyright owner(s) are credited and that the original publication in this journal is cited, in accordance with accepted academic practice. No use, distribution or reproduction is permitted which does not comply with these terms.
*Correspondence: Veronica F. Guwela, v.guwela@gmail.com; Julie King, julieking@nottingham.ac.uk