Feeding on the smallest cells: an in situ study of picoplankton capture by bivalve molluscs from oligotrophic waters
- 1Porter School of Environmental and Earth Sciences, Tel Aviv University, Tel Aviv, Israel
- 2Faculty of Marine Sciences, Ruppin Academic Center, Michmoret, Israel
- 3School of Zoology, George S. Wise Faculty of Life Sciences, Tel-Aviv University, Tel Aviv, Israel
- 4Department of Civil and Environmental Engineering, Technion, Haifa, Israel
- 5Department of Marine Sciences, University of Connecticut, Groton, CT, United States
- 6Laboratoire de Biologie Marine, Université de Nantes, Nantes, France
Introduction: Bivalve molluscs are among the most prominent coastal benthic-suspension-feeders and their farming is the largest and fastest-growing sector of aquaculture. More than a century of intensive laboratory studies (but surprisingly few in-situ studies) has yielded the consensus view that bivalves mainly capture particles >4µm. Nonetheless, bivalves thrive throughout the world’s oceans that are mostly oligotrophic, characterized by low food concentration and dominated by minute autotrophic picoplankton (<2 µm).
Method: We measured, in situ, the capture efficiency of naturally occurring planktonic cells by five suspension-feeding bivalve species from four families and three orders, residing in two oligotrophic basins: the Red Sea and the East Mediterranean Sea.
Results: Three species captured micron and submicron autotrophic cells with high efficiency (60-90%), suggesting a wider trophic niche than hitherto believed. In contrast, two sympatric species captured mainly particles >10 µm.
Discussion: These results suggest that the same basic anatomical tool kit, variably modulated according to taxa, habitat, or life history traits, enables the remarkable evolutionary and ecological success of bivalves in trophically-diverse habitats.
Introduction
The suspension-feeding trophic mode is found in many aquatic taxa, ranging from protists to whales, using a variety of specialized particle-capture and transport mechanisms (Signor and Vermeij, 1994). Bivalve molluscs are the principal suspension feeders in many benthic habitats, and shellfish farming is among the largest and fastest-growing branches of the aquaculture industry (Smaal et al., 2019; Naylor et al., 2021). For these reasons, bivalve feeding has been intensively studied for over a century, mostly in temperate, productive waters characterized by high seston loads and nano and microplanktonic cells, i.e., >2 µm (partially reviewed by Jørgensen, 1966; Ward and Shumway, 2004; Rosa et al., 2018). The knowledge base concerning the feeding mechanisms is incomplete, exceedingly complex, and vigorously debated, comprising dimensions of anatomy and ultrastructure, mucopolysaccharide chemistry, and non-Newtonian fluid mechanics, giving rise to hundreds of scientific papers and treatises (reviewed by Jørgensen, 1966; Jørgensen, 1975; Jørgensen et al., 1988; Ward and Shumway, 2004; Dame, 2013; Gosling, 2015; Rosa et al., 2018).
The bulk of the knowledge of bivalve feeding comes from laboratory studies; however, as noted by Jørgensen (1975): “laboratory data often have restricted value due to uncertainty as to what extent results obtained in the laboratory represent unimpeded activity in nature”. Indeed, in some cases, the feeding rates of bivalves under natural conditions are considerably different from the results obtained in laboratory experiments (Yukihira et al., 2000). Among the many reasons for this discrepancy is that natural food supply fluctuates unpredictably in both quantity and quality and consists of a complex mixture of organic and inorganic particles that are impossible to mimic in the laboratory (Hawkins et al., 1996; Cranford et al., 1998; Riisgård, 2001; Velasco and Navarro, 2005).
Particle size and quality play key roles in capture and feeding (e.g. Cognie et al., 2003; Beninger and Decottignies, 2005). The consensus is that large particles are captured at much higher efficiencies than smaller ones, and the filtration efficiency of most bivalves studied to date sharply diminish for particles< 4 μm (Møhlenberg and Riisgård, 1978; Riisgård, 2001; and see review by Ward and Shumway, 2004; Rosa et al., 2018). Nonetheless, many marine environments, including tropical and subtropical waters, are characterized by low concentrations of food, organic matter, and nutrients, and are dominated by minute autotrophic picoplanktonic cells (< 2 µm) (Jackson, 1980; Berman et al., 1984; Siegel et al., 1989; Falkowski, 2013; Sonier et al., 2016). Such water bodies are often referred to as oligotrophic. Bivalves residing in oligotrophic environments dominated by picoplanktonic cells (< 2 µm) are thus faced with a dual challenge: the paucity of food and its small size. Few studies have addressed feeding characteristics and the strategies employed by bivalves residing in oligotrophic waters (reviewed by Sarà et al., 2003; Yahel et al., 2009).
Israel is located between two of the most oligotrophic and phosphorous-limited marine basins: the Eastern Mediterranean and the Red Sea. Surface chlorophyll concentrations in these basins are usually below 0.5 μg L-1 and rarely exceed 1 μg L-1 (Krom et al., 2014; Shaked and Genin, 2018). Large phytoplankton blooms are rare, picoplankton (<2.0 μm) accounts for the majority of planktonic biomass (Klinker et al., 1978; Krom et al., 1991; Acosta et al., 2013; Ben Ezra et al., 2021) and picoeukaryotic algae account for most of the photosynthetic carbon production in both the Eastern Mediterranean and the Red Sea (Berman et al., 1984; Dishon et al., 2012).
Despite the extreme oligotrophic conditions prevailing in both the Eastern Mediterranean and the Red Sea, bivalves thrive and flourish in both ecosystems (as they do in many oligotrophic systems). For example, the mytilid Brachidontes pharaonis (Fischer, 1870) covers large areas of hard bottom substrate in the intertidal zone and sometimes at greater depths (Rilov et al., 2004). In the subtidal zone, the rock ‘oyster’ Spondylus spinosus accounts for a major part of the macro-invertebrate cover and biomass (Rilov et al., 2004; Yahel and Frid, 2018). Similarly, the pearl ‘oyster’ Pinctada radiata (Leach, 1814), is very abundant in the Levantine Sea (Galil, 2000; Galil and Zenetos, 2002; Lodola et al., 2013). In the Red Sea, the boring mytilid Leiosolenus (Lithophaga) simplex (Iredale, 1939) has a wide range of coral hosts and tens to hundreds of bivalves are commonly found in colonies of different common massive corals (Gohar and Soliman, 1963; Brickner, 1985; Yahel et al., 2009). Another coral borer, the scallop Pedum spondyloideum (Gmelin, 1791) is also a common inhabitant of several coral host species in the Red Sea (Kleemann, 1990). Despite their high abundance, the particle capturing capacities of these dominant species have rarely been studied (but see Yahel et al., 2009).
Measurements of the capture efficiency of bivalves residing in oligotrophic waters are scarce. Clear exceptions are Pouvreau et al. (1999) who directly measured the capture efficiency of Pinctada margaritifera (Linnaeus, 1758) from oligotrophic water and reported values that ranged from 15% for 1 µm particles to 98% for 5 µm particles; and Yahel et al. (2009) that measured in situ the capture efficiency of L. simplex and reported much higher efficiency (69%) for Synchococcus cells slightly smaller than 1 µm. The ecophysiology of B. pharaonis has been intensively studied by Sarà (2006) and Sarà et al. (2000); Sarà et al. (2003); Sarà et al. (2008) in the western Mediterranean Sea, mostly under controlled laboratory conditions and with no reference to their capture efficiency. In more productive waters, Riisgård (1988) studied the capture efficiency of B. exustus (Linnaeus, 1758) in the lab and found low (<50%) efficiency for particles smaller than 4 µm. The feeding of Spondylus limbatus (Sowerby, 1847) juveniles has been studied in the lab using cultured microalgae larger than 3 µm (Marquez et al., 2019). Mathieu-Resuge et al. (2019) used stable isotopes and fatty acids to investigate the diet of Spondylus crassisquama (Lamarck 1819), with no relation to the capture capacity.
To better understand how bivalves thrive in seemingly food-deprived environments dominated by picoplanktonic cells, we investigated in situ, the ability of five common bivalve species residing in the Red Sea and Eastern Mediterranean Sea to capture naturally-occurring cells ranging in size from 0.2 to ~40 µm. To avoid the potential bias associated with laboratory studies, especially for coral boring bivalves (e.g., L. simplex and P. spondyloideum) and bivalves residing in dense macroalgal beds (e.g., S. spinosus), the improved InEx technique of Morganti et al. (2016) was used to study the feeding of undisturbed animals in their local habitat. The present study therefore aimed to elucidate and frame previously unknown aspects of bivalve feeding in oligotrophic environments dominated by picoplanktonic cells.
Materials and methods
Terminology
For the purposes of the present study, microplankton was defined as cells >20 µm, nanoplankton as cells between 2.0 - 20 µm, and picoplankton as cells between 0.2-2.0 µm (Sieburth et al., 1978).
Study site
On the Israeli coast of the Eastern Mediterranean, sampling was conducted via SCUBA at 10-m depth, on a rocky ridge 800 m west of the Michmoret Campus of the Faculty of Marine Science, Ruppin Academic center (32°24’N, 34°51’E) during May-June and October-November of 2016 and 2019. At least 10 individuals were assessed for each of three bivalve species: the mytilid Brachidontes pharaonis (P. Fischer, 1870), the spondylid Spondylus spinosus (Schreibers, 1793), and the Lessepsian-migrant pearl ‘oyster’ Pinctada radiata (Leach, 1814), representing three different orders (Mytilida, Ostreida, and Pectinida, respectively).
At the Northern Red Sea site, sampling was conducted at 12-m depth via SCUBA on a coral reef next to the Interuniversity Institute of Eilat (IUI, 29°30’N, 34°55’E). A total of forty individuals of the boring mytilid Leiosolenus simplex (Iredale, 1939) (order: Mytilida) from two coral host species, Astreopora myriophthalma (Lamarck, 1816) and Goniastrea pectinata (Ehrenberg, 1834), were sampled during November-December 2017. Thirteen individuals of the coral scallop Pedum spondyloideum (Gmelin, 1791) were sampled during July 2021.
Environmental parameters
Temperature of the ambient water was measured every 30 seconds during the sampling sessions, using a PT100 platinum resistance thermometer connected to a FireSting®-O2 system (PyroScience, Germany) that was encased in a custom-made housing. Water samples to assess particulate organic carbon (POC) concentration, were collected using Niskin bottles and processed following Knap et al. (1996). Briefly, samples were filtered immediately in the laboratory (< 2 hr). Each sample was filtered onto a precombusted (2 hours at 350°C) 25-mm GF/F filter (Whatman, Cat. No. 1825-021) prefiltration with 100-µm nylon mesh) using a low vacuum. Blank pre-combusted filters with no samples were used as negative controls. Filters were acidified with fuming HCl to remove all inorganic carbon, dried at 60°C, cut into halves, and stored in tin or silver capsules at -80°C. Samples were analyzed for carbon content using a PDZ Europa ANCA-GSL elemental analyzer interfaced to a PDZ Europa 20-20 isotope ratio mass spectrometer (Sercon Ltd., Cheshire, UK), at the Stable Isotope Facility at the University of California Davis. The contribution of live particulate organic carbon to total organic carbon was estimated by multiplying the cell concentrations (see below) of each prey population (i.e., picoeukaryotic algae, nanoeukaryotic algae, cyanobacteria and non-photosynthetic bacteria) by the conversion factors (carbon content per cell) provided in Table 2 of Houlbrèque and Ferrier-Pagès (2009). Salinity and chlorophyll concentrations in the ambient water were obtained from the local monitoring programs: Israel National Monitoring Program at the Gulf of Aqaba, (http://.iui-eilat.ac.il/Research/NMP) and the Ruppin’s Estuarine and Coastal Observatory at the Mediterranean Sea (RECO, http://reco.ruppin.ac.il/). Both monitoring stations are<1 km from the work sites. Environmental parameters for each sampling site and season are provided in Table 1.
In situ sampling
The diet composition of the bivalves was investigated in situ. Bivalve species, numbers, and locations are summarized in Table 2. For each bivalve, paired samples of inhaled and exhaled water were collected to assess diet composition, using a modified and improved InEx method (VacuSip, Morganti et al., 2016), which is based upon simultaneous, precise, and controlled collection of the water inhaled and exhaled by suspension feeders without interfering with the animals (Figure 1). Use of the InEx technique ensured that only the first step in the feeding process was targeted for measurement, namely, particle capture (Ward and Shumway, 2004; Yahel et al., 2009).
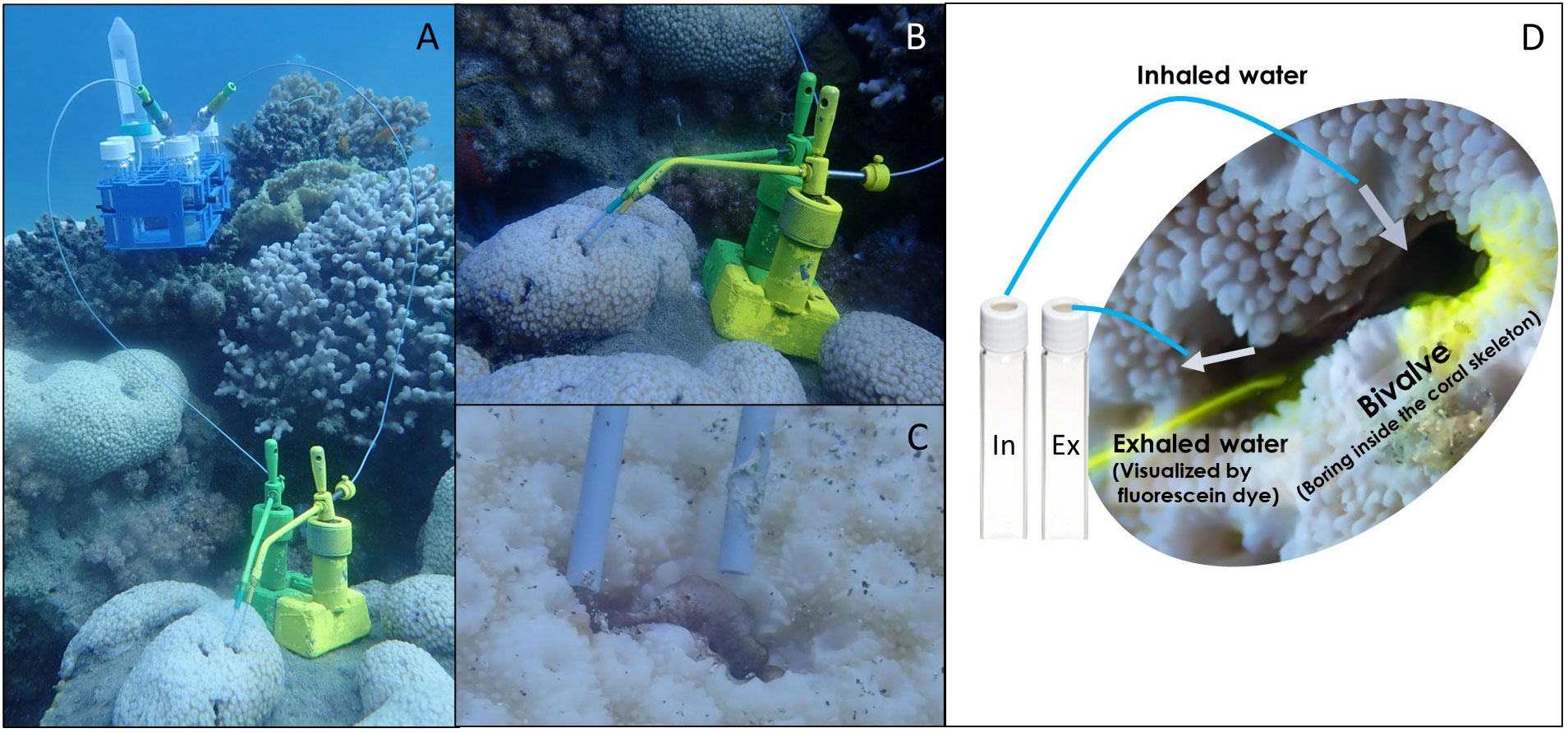
Figure 1 The VacuSIP apparatus used for direct in situ sampling of the water inhaled and exhaled by bivalves. (A) The full experimental setup used for the measurement of the diet composition of the coral-boring bivalve Leiosolenus simplex (siphon diameter<3 mm). (B) The tubes were positioned using custom-made micromanipulators, allowing for minute movements and exact positioning within the inhalant and exhalant jets. (C) A close-up of the sampling tubes positioned in the bivalve-generated inhalant and exhalant jets. (D) A generalized scheme of in situ sampling. Photos: a - c: Rei Diga, d: Shahar Chaikin.
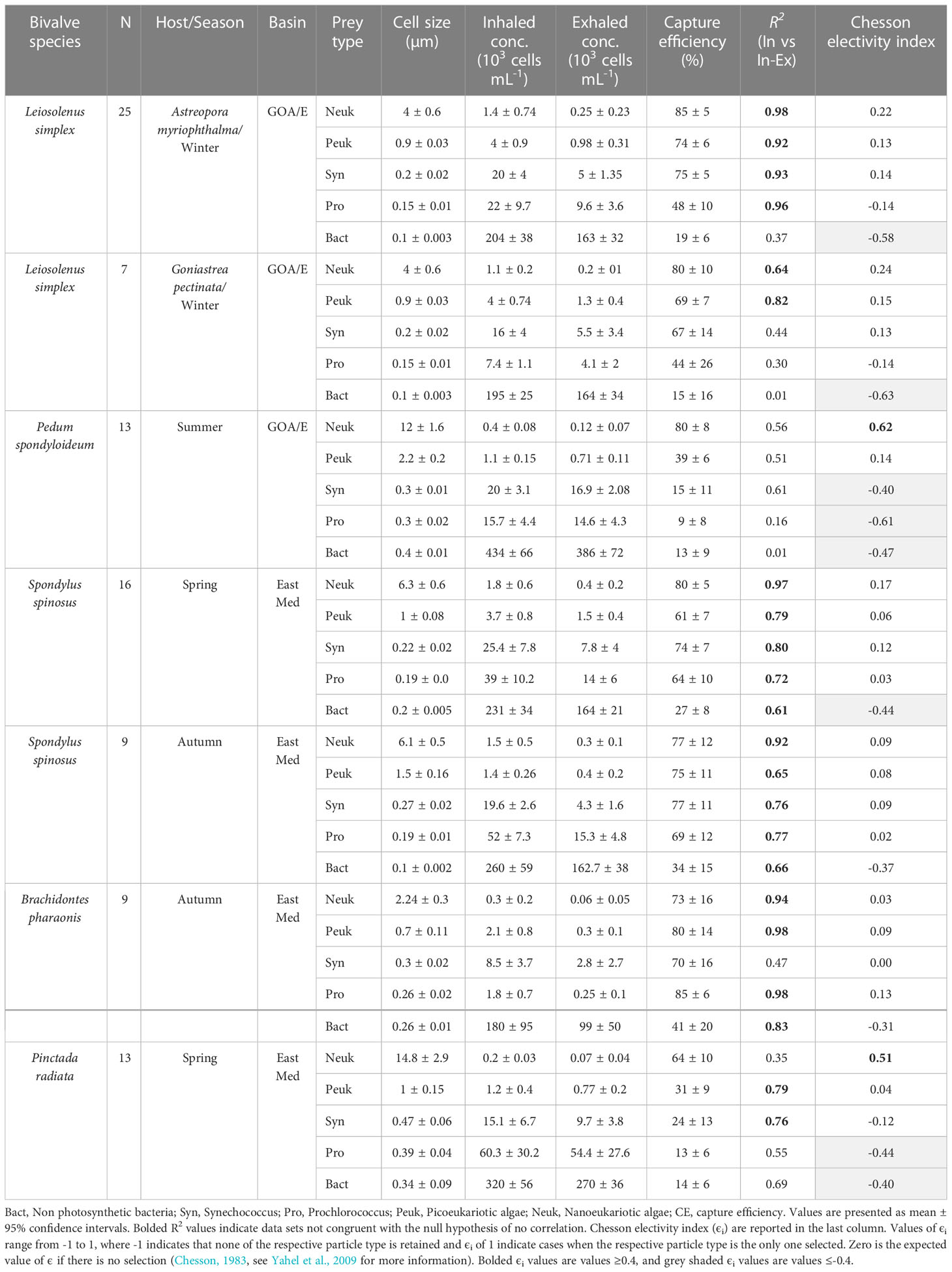
Table 2 Information for each species of bivalve in the study and number of individuals sampled, along with data for each prey taxa identified, including mean concentrations, FCS sizes of cells (the median forward scatter of the respective cell type normalized to the forward scatter of 1-μm polystyrene microspheres) in the inhaled and exhaled water; mean capture efficiency; and R2 for the linear regression of the number of cell captured from each mL of water pumped (calculated as the concentration difference between the inhaled and exhaled water) and prey concentration in the inhaled water.
To ensure that sampled individuals were active and that the sampling tubes were appropriately positioned, the pumping activity of each specimen was visualized before and after sample collection. Fluorescein-dyed seawater was gently released approximately 2 cm from the inhalant aperture of the test specimen, using a syringe equipped with a filter. The speed and magnitude of the exhalant jet provided a clear indication of the pumping activity (Yahel et al., 2005). After ensuring that the sampled specimen was active and filtering (Figure 1D), 5-mL water samples were collected by carefully positioning minute PEEK (polyetheretherketone) tubes (external diameter 1.6 mm, internal diameter 0.27 mm, IDEX Cat. No.1531) inside the exhalant opening and next to the inhalant opening of the sampled bivalve. The tubes were positioned using custom-made manipulators and water suction was initiated by piercing the septum of an evacuated 10.5 mL test tube (Greiner BioOne 455007). The external pressure forced water into the vessel through the sampling tube, and the tube’s small inner diameter ensured a slow and controlled sampling rate. The sampling rate was adjusted to ~1 mL min-1, ensuring integration of the feeding activity and the inherent patchiness of plankton concentrations over several minutes. This process allowed for the simultaneous collection of pairs of inhaled and exhaled water samples and thus minimized sampling biases and errors, as the paired samples were subjected to identical conditions, handling, and analytical processes.
Flow cytometry
The concentrations and characteristics of non-photosynthetic bacteria and four dominant autotrophic groups [Prochlorococcus (Pro), Synechococcus (Syn), picoeukaryotic and nanoeukaryotic algae (PEuk and NEuk)] were analyzed using an AttuneNXT® acoustic focusing flow cytometer (Applied Biosystems) equipped with a syringe-based fluidic system that allowed precise adjustment of the injected sample volume and hence high precision of the measured cell concentrations (± 5%). The optical system contained violet and blue lasers (405 and 488 nm, respectively). Duplicates of 1.8 mL were collected from each water sample and transferred into 2-mL cryovials (Corning, Cat. No. 430659). Samples were incubated for at least 15 min at room temperature (~25°C) with 0.1% glutaraldehyde (final concentration) using 50% electron microscopy grade glutaraldehyde (Sigma-Aldrich, Cat No. 340855), frozen in liquid nitrogen (> 60 min), and then stored at -80°C until analyzed (within one month).
Each water sample was analyzed twice. First, 600 µL of the sample were analyzed at a high flow rate (100 µL min-1) to determine the presence of pico and nano-phytoplankton with a dual threshold (trigger) on the red-fluorescence channels of the violet and blue lasers. A second analysis was used to detect cells with no autofluorescence, i.e., non-photosynthetic microbes. To visualize these cells, a 300-µL aliquot of the water sample was incubated with the nucleic acid stain SYBR Green I (20-120 min dark incubation at ~25°C, 1:104 dilution of the SYBR Green commercial stock). For this analysis, a low flow rate of 25 µL min-1 was used and the instrument was set to high sensitivity mode. A 75-µL sample was analyzed with a dual threshold (trigger) on green-fluorescence channels of the violet and blue lasers. Taxonomic discrimination was made based upon orange fluorescence (Bl2, 530 ± 30 nm) of phycoerythrin and red fluorescence (Bl3, 695 ± 40 nm) of chlorophyll. Side-scatter (SSC) was used as a proxy of cell surface complexity and cell volume, and forward-scatter (FSC) was used as a proxy of cell size (Jacobi et al., 2021 and references therein). Given the very weak chlorophyll fluorescence of Prochlorococcus in near-surface waters, especially in summer, full separation of their population from the noise signal was not always possible. Reference microspheres (Polysciences™, Cat. No. 23517, Flow Check High-Intensity Green Alignment 1.0 µm) were used as an internal standard in each sample (Dadon-Pilosof et al., 2017).
Data analyses
Data files from flow cytometric measurements were processed with “FCS express 4” software (De-Novo™), to quantify the nano- and pico-eukaryotic algae, Prochlorococcus, Synechococcus, and non-photosynthetic bacteria. The median size of each planktonic cell population was estimated as the ratio of the median forward scatter () of the respective planktonic cell population (i) to that of the median forward scatter of standard 1-µm non-fluorescent polystyrene spheres (, Molecular probes, Cat No. F13838, F13839) that were included in each flow cytometry run: Because the refraction index of the polystyrene calibration spheres differs from that of planktonic cells by an unknown extent, it is possible that the FCS size deviates somewhat from the true cell size (Foladori et al., 2008), especially for the smaller, sub-micron groups (i.e., non-photosynthetic bacteria, Prochlorococcus, and Synechococcus). These size estimations are thus termed ‘FCS size’ in the present work: The mean ( ± confidence interval of 95%) of the median value of the forward scatter of all particles in each defined group in each inhaled water sample. It should be noted that the size distribution of each group is usually very wide, as exemplified in Figures 2C, D of Jacobi et al. (2018).
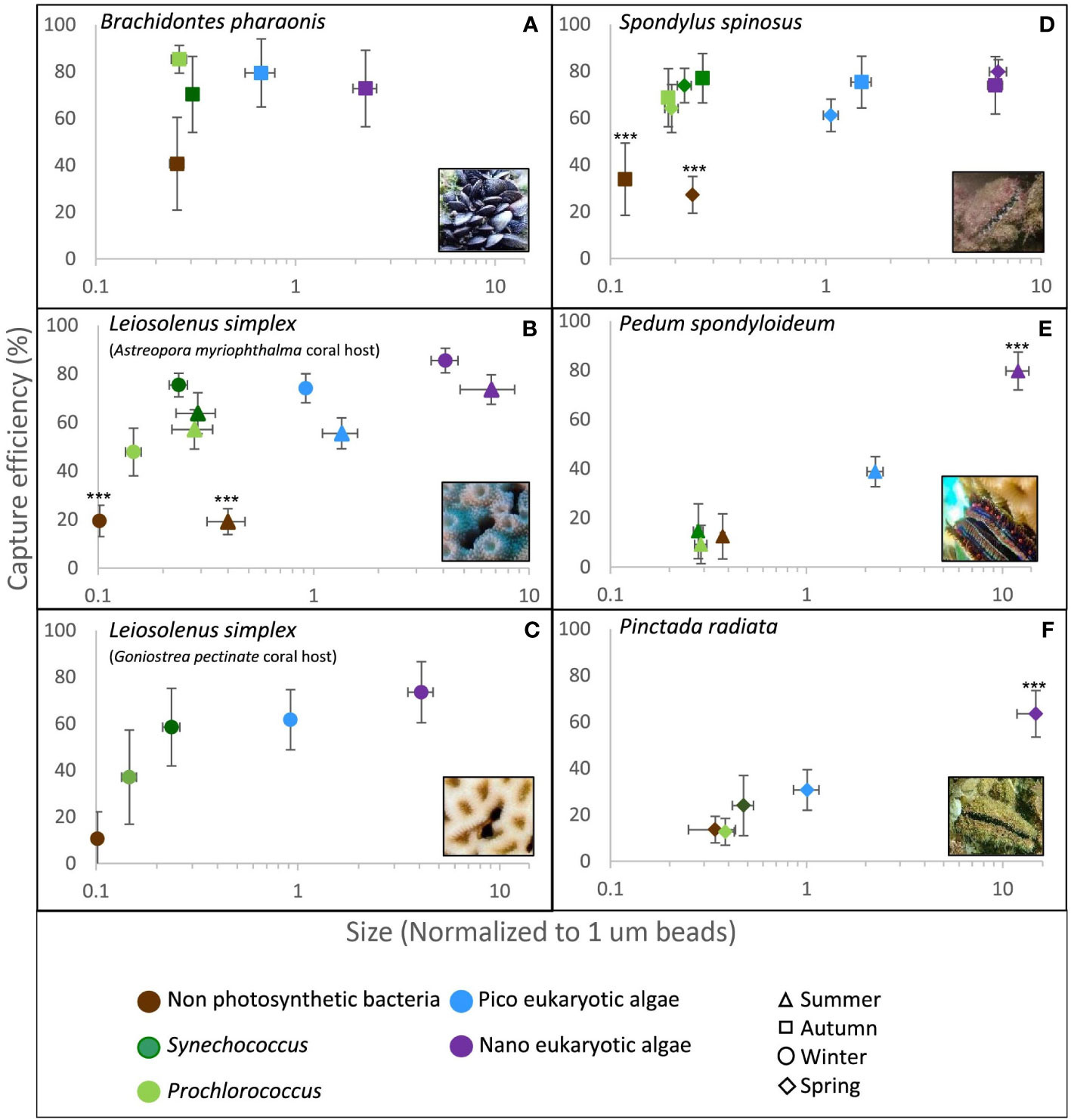
Figure 2 Capture efficiency of the dominant planktonic groups by five different bivalve species as measured in situ at the Gulf of Eilat/Aqaba, Red Sea and at the Eastern Mediterranean Sea. The median forward scatter (proxy of cell size) of each group was normalized to the forward scatter of 1.0 µm reference spheres (X axis, note the logarithmic scale). Error bars are 95% confidence intervals. Asterisks indicate statistically significant differences (i.e., data sets not congruent with the null hypothesis of no difference with respect to all other data sets) in capture efficiency of the planktonic group from all other groups (***p<0.001, Repeated Measures ANOVA). (A) Brachidontes pharaonis (n=9); (B) Leiosolenus simplex from Astreopora myriophthalma host during winter (n=25) and summer (n=12); (C) Leiosolenus simplex from Goniastrea pectinata host (n=7); (D) Spondylus spinosus during spring (n=16) and autumn (n=9); (E) Pedum spondyloideum (n=11); (F) Pinctada radiata (n=17).
The capture efficiency of each planktonic group by individual bivalves was calculated as
where CE is the capture efficiency, and Cin, and Cex are the concentrations of a given planktonic group in the inhalant and exhalant water, respectively. To avoid the potential bias associated with collecting water samples from bivalves that stopped pumping during the sampling period, and thus contaminating those samples with ambient water, paired water samples that did not exhibit at least 50% of the highest capture efficiency measured for any of the planktonic groups were eliminated from analysis. This procedure resulted in elimination of ~ 10% of the paired water samples collected (Table S2). Chesson’s selectivity (αi) and electivity (ϵi) indices (Chesson, 1983) were calculated as described in Yahel et al. (2009), using the mean capture efficiencies for each planktonic group.
Much of the present study was necessarily observational in nature, since the previous knowledge base was virtually nonexistent, rendering hypothesis testing impossible at this point (see Beninger et al., 2012 for review). Because all measurements were conducted in situ, we consider the results as reliable reflection of the natural variability of the activity of the bivalves during the sampling periods. It should be noted that the focus of this study was the comparison of the capture efficiencies for different prey types within each bivalve species. As not all bivalve species were sampled in the same location, time, or ocean, the comparison between the capture efficiencies of the different bivalve species should be considered with caution.
A “within subject” design (i.e., Repeated Measures Analysis of Variance [ANOVA], and its nonparametric alternative: Friedman ANOVA on Ranks), was used to test the null hypothesis of nonselective retention of the different cell types. To meet the assumptions of normality and homogeneity of variance (tested with Levene’s test), capture efficiency data (%) was logit transformed and analyzed using Repeated-Measures ANOVA with the five prey types (i.e., Nanoeukaryotic algae, Picoeukaryotic algae, Prochlorococcus, Synechococcus, and non-photosynthetic bacteria) as repeated measures (within-subject variables). Differences in the capture efficiency of different-sized planktonic cells by the bivalves were tested using non-parametric tests since the data violated the assumption of homogeneity of variance. Data are reported as mean values ± 95% confidence intervals, unless stated otherwise. A significance level of α = 0.05 was used in all analyses.
Results
Environmental conditions
The Gulf of Eilat/Aqaba (GoE/A)
Temperature remained very stable over the sampling sessions, with mean (± 95% confidence intervals) values of 23.4 ± 0.003°C during the 2017 winter sampling session and 26.7 ± 0.05°C during the 2021 summer session. The maximum chlorophyll a concentration was 0.6 µg L-1, with a mean value of 0.5 ± 0.02 µg L-1 during the winter of 2017, and 0.20 ± 0.05 µg L-1 during the summer of 2021. The planktonic community was numerically dominated by picoplanktonic cells, comprising >99% of the community in all ambient water samples. The ambient concentration of the rare nanoeukaryotic algae (<0.6% of the community), with a mean FSC size of 4.1 ± 0.6 µm, ranged between 0.6 x 103 to 6.9 x 103 cells mL-1 (Table 2; Figure 3). Within the picoplankton community, the minute non-photosynthetic bacteria, accounted for most of the planktonic cells (> 80% of cell counts). The majority of the pico-phytoplanktonic community during 2017 was composed of Prochlorococcus (18.9 x 103 ± 7.9 x 103 cells mL-1, mean ± confidence intervals), and Synechococcus (19 x 103 ± 3.4 x 103 cells mL-1, Table 1).
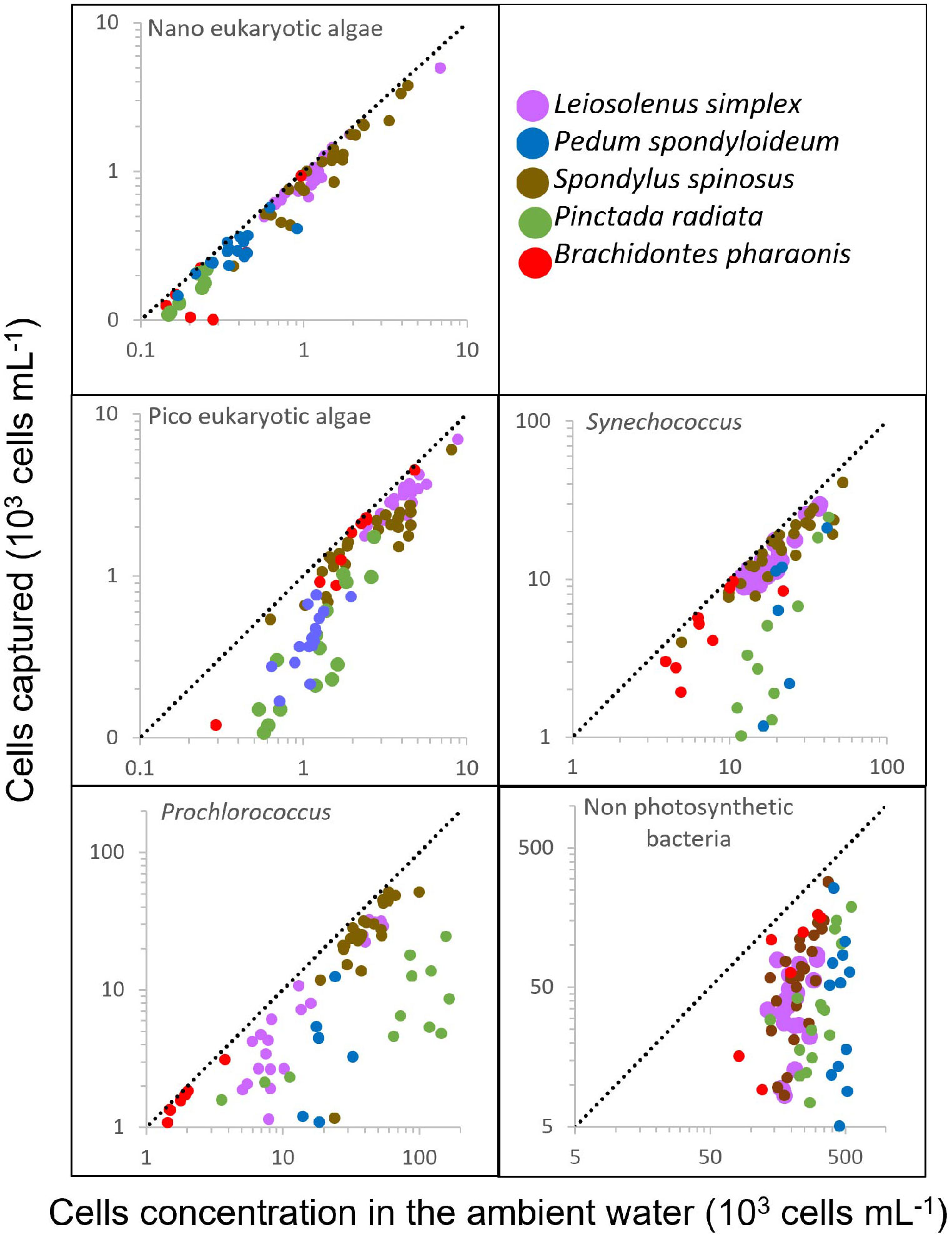
Figure 3 Bivalve response to variation in prey concentration in oligotrophic water. The number of captured cells per mL of water pumped (calculated as the difference between the inhalant and exhalant concentrations) is plotted against the cell concentrations in the ambient (inhaled) water. Different bivalve species are indicated by different colors. Dashed lines represent slope=1; i.e., 100% capture efficiency. Number of samples and Regression R2 are indicated in Table 2.
East Mediterranean Sea
Water temperature fluctuated more in the East Mediterranean sites compared to the Red Sea site, with values during the 2016 and 2020 spring sampling sessions ranging between 22.0 to 23.0°C, while values during the 2016 and 2020 autumn sampling sessions ranged from 26.1 to 27.4°C and 26.8 to 28.2°C, respectively. Apart from spring 2016, in which the average chlorophyll a concentration was 1.0 µg L-1, the chlorophyll concentration was less than 0.4 µg L-1 during all sampling sessions (Table 1). Concentrations of particulate organic matter in the ambient water in the East Mediterranean Sea site were two times higher than those measured in the Red Sea (Table 1), with a mean concentration of 8.6 ± 3.5 µM and 9.4 ± 2.02 µM for spring and autumn, respectively; however, phytoplankton accounted for less than 20% of this matter. The planktonic community during all sampling sessions was composed of >99% picoplanktonic cells, of which 22-32% were phototrophic cells (Table 2). The size of these cells differed between sampling seasons (Table 2). Non-photosynthetic bacteria, with a mean FCS size of 0.1 to 0.2 µm (spring and autumn, respectively) accounted for >75% of the community in both seasons during 2020.
Both study sites were exposed to ambient water from the open sea and experienced continuous water exchange. Sampling was conducted under low-medium currents, usually in the range of 0.05-0.1 m sec-1 (T. Amit, unpublished data).
Particle capture efficiency
Rare nanoeukaryotic algae were captured efficiently (>60%) by all bivalve species. The spondylid Spondylus spinosus and the two mytilids, Leiosolenus simplex and Brachidontes pharaonis, exhibited high capture efficiency for picophytoplankton cells (smaller than 2.0 µm), removing on average 67 to 77% of the ~1.0 µm (FSC size) Synechococcus cells, and 61 to 80% of the picoeukaryotic algae (Table 2; Figures 2A–D). In contrast, the pearl ‘oyster’ Pinctada radiata and the scallop Pedum spondyloideum were much less efficient at capturing picophytoplankton cells, removing less than 24% of the Synechococcus cells and less than 39% of the picoeukaryotic algae (Figures 2E, F). Non-photosynthetic bacteria were captured at lower efficiency by all bivalves (15-41%) despite their numerical dominance in the plankton, and despite having a comparable size range to that of some photosynthetic groups (Table 2; Figure 2). Nonetheless, it should be noted that S. spinosus and B. pharaonis captured more than 25% of this particle type. These trends remained similar between different sampling periods despite seasonal differences in both size and concentration of the different prey taxa.
Particle capture efficiency by L. simplex from both coral host species, Astreopora myriophthalma and Goniastrea pectinata, did not differ significantly with respect to prey taxa or season (Figures 2B, C). However, L. simplex living in the A. myriophthalma host captured significantly lower amounts of non-photosynthetic bacteria. Both species of bivalve captured the picoeukaryotic algae and Synechococcus at >65% efficiency and exhibited lower efficiency (<48%) for the smaller Prochlorococcus cells (Table 2; Figures 2B, C). The mussel B. pharaonis removed the minute pico-cyanobacterium Prochlorococcus with high efficiency (85 ± 6%) and the similar FCS size pico-cyanobacterium Synechococcus with an efficiency of 70 ± 16%.
Similarly, the two mytilids and the spondylid S. spinosus captured all phototrophic cells, including those< 2.0 µm, at greater than 60% efficiency (Figure 2D). Nanoeukaryotic algae were rare and were captured with more than 75% efficiency. The non-photosynthetic bacteria were captured with the lowest efficiency (27 ± 8% and 34 ± 15% for spring and autumn, respectively; Table 2).
Compared to the other species of bivalves studied, the coral-dwelling scallop P. spondyloideum exhibited the lowest capture efficiency for all picophytoplankton groups, capturing Prochlorococcus, for example, at an efficiency of only 9 ± 8%. The highest capture efficiency (80 ± 8%, Table 2; Figure 2E) was recorded for the nanoeukaryotic algae. The pearl oyster, Pinctada radiata, showed capture efficiency similar to that of Pedum spondyloideum, capturing rare nanoeukaryotic algae with 64 ± 10% efficiency, whereas all smaller cells (<2.0 µm) were captured with less than 31% efficiency (Figure 2F). In contrast to the other bivalve species in this study, it was not possible to reject the null hypothesis of non - selective retention of the Prochlorococcus and the non-photosynthetic bacteria.
Relationship between prey concentration and cell capture
To examine the relationship between prey concentration and cell capture, we plotted, for each prey type, the number of cells removed from each mL of exhaled water versus the concentrations of prey cells in the ambient (inhaled) water (Figure 3). It should be noted that these values are independent of the pumping rate. For most prey types and bivalve species, the capture efficiency of phytoplanktonic cells was constant and independent of their concentration in the ambient water (F test for linear regression, degrees of freedom of the residuals ranged from 5 to 23, pending on the taxa; see Table 2 for more details). In contrast, for the oyster P. radiata the capture efficiency of Synechococcus, picoeukaryotic, and nanoeukaryotic algae increased with an increase in ambient concentrations.
Discussion
In situ capture of picoplankton
The data of the present study demonstrates the ability of the studied, undisturbed bivalves to capture picoplankton at high efficiency (41-85%) in their natural habitat. Two mytilids (Brachidontes pharaonis and Leiosolenus simplex) and the spondylid Spondylus spinosus captured picoplanktonic cells (<2.0 µm) with high efficiency (>60%), as previously demonstrated by Yahel et al. (2009) at the same sites as the current study, providing a rare demonstration of true replication in marine ecology (Johnson, 1999; Kruschke, 2010; Beninger et al., 2012). Although sampling and analyses were conducted using slightly different methods, the authors measured similar capture efficiency for the small photosynthetic bacteria Synechococcus and Prochlorococcus of 69% (± 14 SD) and 41% (± 19 SD), respectively (Yahel et al., 2009).
In contrast, the coral scallop Pedum spondyloideum, which shares the same habitats with L. simplex, and the pearl ‘oyster’ Pinctada radiata, which shares the same habitats with B. pharaonis and S. spinosus, captured picoplanktonic cells at a much lower efficiency. This finding suggests that different bivalve taxa may be utilizing different feeding strategies/mechanisms in oligotrophic waters dominated by extremely small particles.
The mussel B. pharaonis is a successful invader in the eastern Mediterranean Sea that displaces and eliminates native species from their habitats (Rilov et al., 2004; Rilov, 2009). In an extensive series of experiments (Sarà et al., 2000; Sarà et al., 2003; Sarà, 2006; Sarà et al., 2008), it was shown that B. pharaonis can assimilate a wide range of foods (Sarà, 2006) and exploit multiple trophic levels (Sarà et al., 2003; Sarà et al., 2008). The results presented here support this conclusion, as B. pharaonis had the highest capture efficiency for picoplanktonic cells, including non-photosynthetic bacteria, of all studied species.
It should be noted that the sampling methods used in the current study are sensitive to factors that could introduce biases. If the bivalve ceases pumping momentarily during sampling, or if the tube collecting the exhalant water is positioned in such a way that the exhalant sample becomes ‘contaminated’ with ambient seawater, the resulting calculated capture efficiency will be underestimated. With this in mind, capture efficiency measured in this study never reached 100%, even for cells > 10 μm (Figure 2E, Table 2). In contrast, available data demonstrate that most bivalves capture particles greater than 6 μm at close to 100% efficiency (Rosa et al., 2018). This discrepancy suggests that the samples in the current study may have been ‘contaminated’ to some extent with ambient water, thus the capture efficiency values reported here are underestimated. Alternatively, the data presented here, obtained by direct sampling underwater, may suggest that under field conditions, bivalves rarely reach 100% efficiency, even for nanoeukaryotic algae.
Surface chlorophyll concentrations in both the Red Sea and the East Mediterranean Sea are usually below 0.5 μg L-1 (Krom et al., 2014; Shaked and Genin, 2018), and large phytoplankton blooms are rare and short lived (Al-Najjar et al., 2007). While the contribution of such rare events to the diet of bivalves can be substantial (McCammon, 1969; Cowen, 1971), its quantification is challenging, due to the sporadic and short-lived nature of these events. While temperature can vary widely in both studied habitats, during the sampling sessions temperature was stable, and ranged between 22-28°C across all sampling sessions and sites (Table 1). It is therefore unlikely that variation in temperature or salinity had a significant effect on the variation in the measured capture efficiency.
Modulation of picoplankton capture
The results of the present study suggest that, as previously reported (Yahel et al., 2009), size is not the only factor determining capture efficiency, since all studied bivalves captured non-photosynthetic bacteria at lower efficiency than similar-size phototrophic picocyanobacteria (Synechococcus and Prochlorococcus). In fact, an important effect of particle surface properties on the capture efficiency of bivalves has been previously reported (Hernroth et al., 2000; Riisgård and Larsen, 2010; Rosa et al., 2013; Dadon-Pilosof et al., 2017; Rosa et al., 2017), especially in the smaller size range (Rosa et al., 2018). Dadon-Pilosof et al. (2019) and Jacobi et al. (2021) reported a similar phenomenon for several tunicates and suggested that some planktonic cells have a non-sticky cell surface, which may enable them to evade capture by suspension feeders.
In the eastern Mediterranean and in the GOE/A, bivalve species with overlapping distribution and habitats were sampled (Kleemann, 1990; Mienis et al., 1993; Galil et al., 2013; Shabtay et al., 2015; Diga et al., 2022). At the Red-Sea coral reef, P. spondyloideum and L. simplex share the same habitat, burrowing inside live coral hosts, and often can be found adjacent to each other in the same host colony (T. Amit and G. Yahel, personal observations). Similarly, on the rocky coast of the Mediterranean Sea, P. radiata can be found throughout the littoral zone, where it shares its habitat with B. pharaonis (Diga et al., 2022). In the subtidal zone, it also can be found attached to the shells of S. spinosus (T. Amit, personal observations). The size spectrum and the electivity index of the particles captured by Pedum spondyloideum and Pinctada radiata was, however, considerably different than that of neighboring L. simplex, B. pharaonis, and S. spinosus. The ability of L. simplex, B. pharaonis, and S. spinosus, but not Pedum spondyloideum and Pinctada radiata, to capture the most abundant picoplanktonic cells suggests a trophic niche separation between the species. Whereas L. simplex, B. pharaonis, and S. spinosus can utilize the smallest and most abundant cells, the sympatric species Pedum spondyloideum and Pinctada radiata, respectively, cannot, as can also be seen from the large differences in electivity indices (Table 2). Thus, these species may rely upon different physiological strategies such as elevated filtration (pumping) rate (Hawkins et al., 1998; Pouvreau et al., 1999), the capture of rare, larger, and more nutritious cell aggregates (Kach and Ward, 2008; Ward and Kach, 2009), better usage of coral-derived organic matter (Shafir and Loya, 1983; Naumann et al., 2010), and/or reduced metabolic rate (Riisgård et al., 2003; Sokolova et al., 2012).
For Pedum spondyloideum and Pinctada radiata, capture efficiency of picophytoplankton increased as cell concentrations increased. A concentration-dependent efficiency was also demonstrated by Palmer and Williams (1980) for the bay scallop Argopecten irradians for particles of 1.7 to 3.4 µm. The authors suggested that the change in capture efficiency was a result of an increase in mucus production by the ctenidia of the scallop. Both Pedum spondyloideum and Pinctada radiata may be adjusting their capture process to intercept the smaller picoplankton only when the concentration of these cells is high enough to compensate for the energetic expenditure required for such adjustments and maintenance.
Comparison with picoplankton capture by bivalves from productive habitats
The comparison presented in Figure 4 underscores the paucity of published data regarding the capture efficiency of submicrometer cells. It also shows the high efficiencies with which picoplanktonic cells were captured by some, but not all, of the bivalve species in the current study. Finally, the comparison demonstrates the low efficiencies with which all studied bivalves captured the non-photosynthetic picoplanktonic cells (i.e., ‘Bact’ in Figure 4) despite the size overlap with the picoplanktonic cells (see also Yahel et al., 2009; Dadon-Pilosof et al., 2017; Dadon-Pilosof et al., 2019; Jacobi et al., 2021), suggesting a pre-capture negative selection with respect to these cell types.
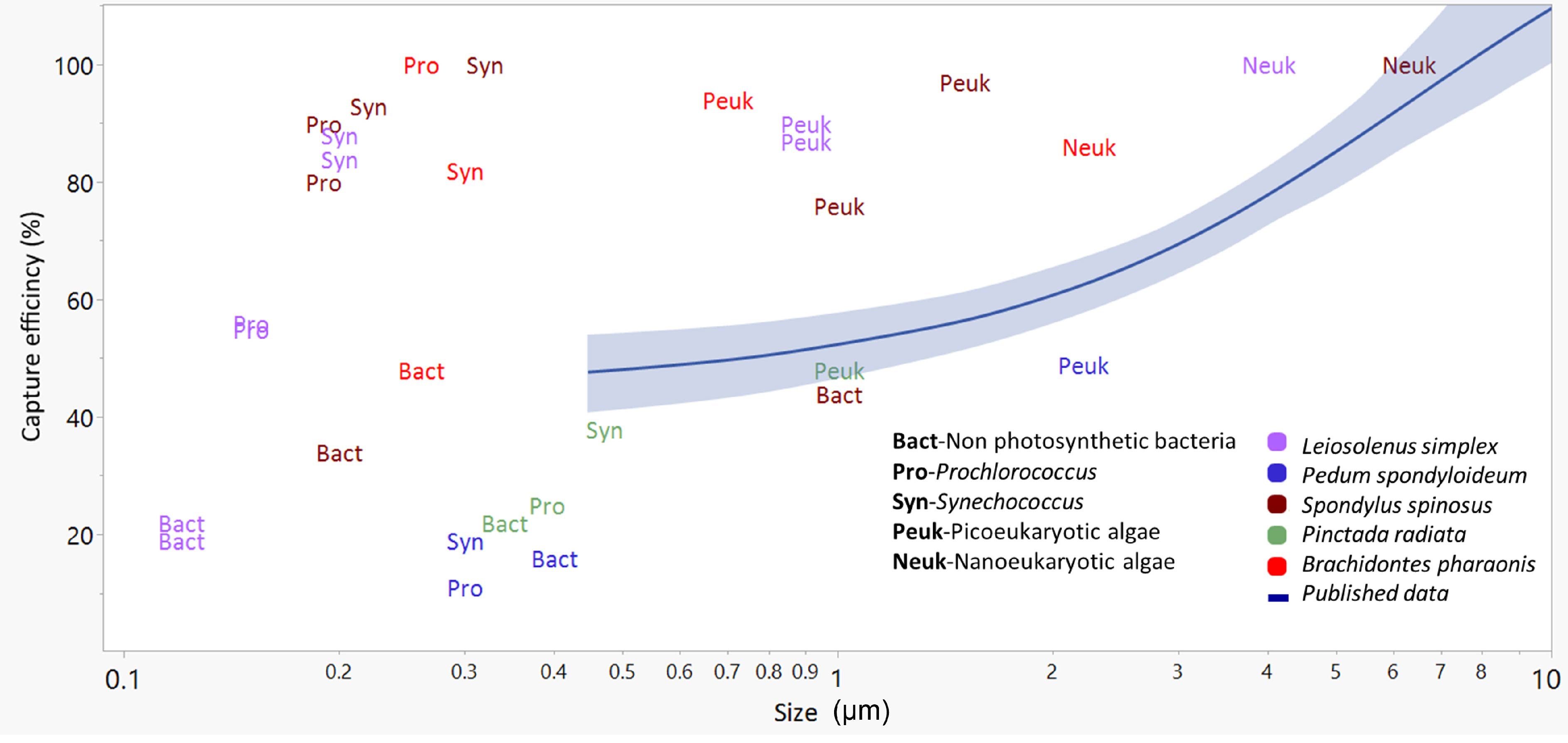
Figure 4 The relationships between the size of small (<10 µm) planktonic cells (X-axis) and their capture efficiency (Y axis) presented as a mean trend line (blue line) with 95% confidence interval (shaded blue) for published data (29 species, mostly from productive waters, see Table S1). Colored labels present data from the current study (5 species). Bact, Non photosynthetic bacteria; Syn, Synechococcus; Pro, Prochlorococcus; Peuk, Picoeukaryotic algae; Neuk, Nanoeukaryotic algae. Prey size was estimated differently in different studies (see Table S1) and is presented here on a logarithmic scale.
Most of the published information on bivalves reports low capture efficiency for particles smaller than 4 µm (Møhlenberg and Riisgård, 1978; Jørgensen et al., 1988; Riisgård, 1988; Ward and Shumway, 2004; Rosa et al., 2018 and see Table S1). In the few cases where high values of capture efficiency of small particles and cells have been reported, outside of tropical or Mediterranean waters, the bivalve species occurred in freshwater or reduced-salinity environments (Riisgård, 1988; Langdon and Newell, 1990; Silverman et al., 1995). Qiao et al. (2022) recently reported high levels of nano- and picophytoplankton in the digestive system of three bivalve species in high-productivity waters. Despite several factual and contextual errors in that publication that are unrelated to this point, their results, along with our own ongoing observations, suggest that efficient capture of small particles by some taxa may be occurring under some conditions in highly productive habitats. An attempt at the reconciliation of these contradictory observations is presented below.
Underlying mechanisms for picoplankton capture
The universality of the basic pallial organs in autobranch bivalves suggests that the same anatomical structures may be used in the optimization of particle capture at the size ranges that are dominant in the normal habitats of the particular bivalve species. It is well established that different bivalve taxa, especially at or above the family level, have different adult gill morphologies and types that enable them to capture different sizes of particles at varying efficiencies (see review by Ward and Shumway, 2004). Presumably, the same types of structures are used in all cases, including: (1) variously configured gills (2) variously-configured cilia (simple, compound, composite, J-cilia lengths, densities, and numbers), and (3) mucus of varying types that relate to function and anatomical context (Beninger et al., 1992; Beninger et al., 1993; Beninger and St-Jean, 1997; Beninger et al., 1997; Beninger et al., 2003). A summary of the available data concerning the taxonomy and gill structure (type of gill and associated ciliary tracts) of the studied species is presented in Table 3. The most efficient capture of small cells was found in the two mytilid species and in the spondylid, while the least efficient was found in the pectinid and margaritid species. It is tempting to conclude that the presence of laterofrontal cirri (compound cilia) is responsible for enhanced capture efficiency of picoplankton, since bivalves that possess such cirri (e.g., mytilids) are known to capture smaller plankton more efficiently in habitats dominated by large particles and cells (Riisgård et al., 1996; Silverman et al., 1999; Riisgård, 2002) compared to bivalves that do not possess these complex structures (e.g., pectinids, margaritids). However, to date there is no published data concerning the status of laterofrontal cirri in spondylids; such information is therefore a high research priority.
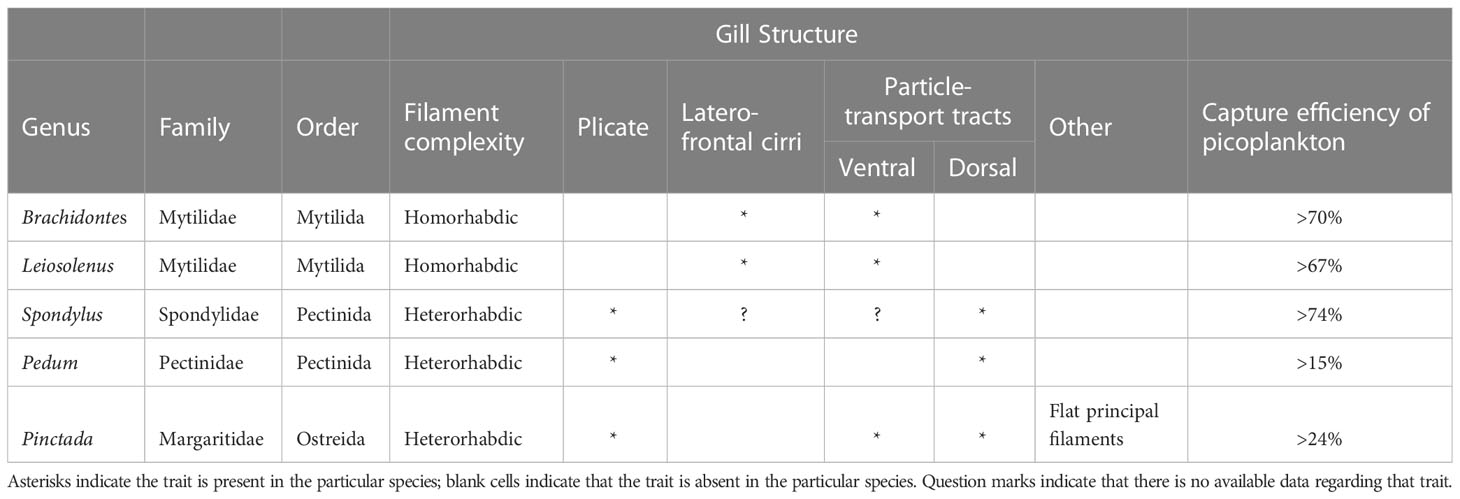
Table 3 A summary of the available data regarding taxonomy and gill structure of the studied species.
The contradiction between the above-mentioned studies showing declining capture efficiency for nanoplankton, and the studies showing strong capture of picoplankton, may be explained by the possible utilization of different capture mechanisms for the two size classes ‘micro-nano’ and ‘pico’: the former being captured mainly by the action of the laterofrontal ciliary tracts in species which possess such tracts (Vahl, 1972; Jørgensen, 1976; Jørgensen et al., 1984; Jørgensen, 1989; Ward et al., 1998). Below the threshold of 3-4 µm, however, this capture mechanism appears to be inefficient for all bivalves tested (Møhlenberg and Riisgård, 1978; Riisgård, 1988; and reviewed by Ward and Shumway, 2004; Rosa et al., 2018). It may be that such particles are simply absorbed onto the abundant mucus present on the gills, and thus represent somewhat ‘incidental’, but nonetheless consequential captures (Beninger et al., 2003; Beninger et al., 2007). With two such available feeding mechanisms, overlap may be expected at the size range interfaces, and some taxa in some habitats may specialize more in one mode than the other.
In the present study, B. pharaonis, L. simplex, and S. spinosus all demonstrated picoplankton capture (<2.0 µm) of natural phytoplanktonic cells at a much higher efficiency than that reported in the literature for bivalves from productive waters that are dominated by nano- and microphytoplankton (Figure 4, Table S1, and references therein). Taken together, the studies cited above, and the findings reported here, suggest that picoplanktonic cells, including those < 1.0 µm, may be a more important planktonic component for some bivalve species than previously considered, especially in oligotrophic environments dominated by small cells. It is noteworthy that while picoplankton numerically dominated the cells captured by some of the bivalves, the biovolume of an e.g., 0.8 µm picoplanktonic cell is ~120 times smaller than that of a 4 µm eukaryotic algae. Therefore, despite the low abundance of microalgae larger than 4 µm, the contribution of microalgae to the bivalves’ diet is substantial. The exact contribution of each cell population to the bivalves’ diet is not reported here due to our limited ability to accurately determine the size of the cells smaller than 1 µm.
The plasticity in capture efficiency arising from the considerations outlined above may enable the different bivalve taxa to thrive as functional groups in native waters of vastly different particulate organic matter characteristics - no doubt contributing to the remarkable evolutionary and ecological success of this group of suspension-feeders.
Data availability statement
The original contributions presented in the study are included in the article/Supplementary Material. Further inquiries can be directed to the corresponding author.
Author contributions
TA, YL, and GY contributed to the conception and design of the study. TA, RM, and YJ conducted the in situ sampling. TA, GY, and PB performed the statistical analysis. TA, GY, YL, PB, SS, and JW contributed to interpreting the results. TA wrote the first draft of the manuscript. All authors contributed to the article and approved the submitted version.
Funding
This work was funded by a grant from a philanthropic fund to the INPA, BSF Grant 2012089 to GY, ISF Grant 640/21 to YL, ISF grant 249/21 to GY, BSF, and NSF IOS grants 2017622 and 1755409 to GY, SS, and JW.
Acknowledgments
We thank the staff of the Faculty of Marine Science in Michmoret (Ruppin Academic Center) and the Inter-University Institute in Eilat (IUI) for support and use of the scientific diving centers and running seawater facilities, the Yahel and Loya lab members for their help in the in situ work, Henk Mienis for help with the identification of bivalve species, Noga Gavriely, Neomi Darmon, and Rei Diga for help with the lab work and analyses.
Conflict of interest
The authors declare that the research was conducted in the absence of any commercial or financial relationships that could be construed as a potential conflict of interest.
Publisher’s note
All claims expressed in this article are solely those of the authors and do not necessarily represent those of their affiliated organizations, or those of the publisher, the editors and the reviewers. Any product that may be evaluated in this article, or claim that may be made by its manufacturer, is not guaranteed or endorsed by the publisher.
Supplementary material
The Supplementary Material for this article can be found online at: https://www.frontiersin.org/articles/10.3389/fmars.2023.1184773/full#supplementary-material
References
Acosta F., Ngugi D. K., Stingl U. (2013). Diversity of picoeukaryotes at an oligotrophic site off the northeastern red Sea coast. Aquat. Biosyst. 9, 16. doi: 10.1186/2046-9063-9-16
Al-Najjar T., Badran M. I., Richter C., Meyerhoefer M., Sommer U. (2007). Seasonal dynamics of phytoplankton in the gulf of aqaba, red Sea. Hydrobiologia 579, 69–83. doi: 10.1007/s10750-006-0365-z
Ben Ezra T., Krom M. D., Tsemel A., Berman-Frank I., Herut B., Lehahn Y., et al. (2021). Seasonal nutrient dynamics in the p depleted Eastern Mediterranean Sea. Deep Sea Res. Part I 176, 103607. doi: 10.1016/j.dsr.2021.103607
Beninger P. G., Boldina I., Katsanevakis S. (2012). Strengthening statistical usage in marine ecology. J. Exp. Mar. Bio. Ecol. 426–427, 97–108. doi: 10.1016/j.jembe.2012.05.020
Beninger P. G., Decottignies P. (2005). What makes diatoms attractive for suspensivores? the organic casing and associated organic molecules of Coscinodiscus perforatus are quality cues for the bivalve Pecten maximus. J. Plankton Res. 27, 11–17. doi: 10.1093/plankt/fbh156
Beninger P. G., Decottignies P., Guiheneuf F., Barillé L., Rincé Y. (2007). Comparison of particle processing by two introduced suspension feeders: selection in Crepidula fornicata and Crassostrea gigas. Mar. Ecol. Prog. Ser. 334, 165–177. doi: 10.3354/meps334165
Beninger P. G., Dufour S. C., Bourque J. (1997). Particle processing mechanisms of the eulamellibranch bivalves Spisula solidissima and Mya arenaria. Mar. Ecol. Prog. Ser. 150, 157–169. doi: 10.3354/meps150157
Beninger P. G., Dufour S. C., Decottignies P., Le Pennec M. (2003). Particle processing mechanisms in the archaic, peri-hydrothermal vent bivalve Bathypecten vulcani, inferred from cilia and mucocyte distributions on the gill. Mar. Ecol. Prog. Ser. 246, 183–195. doi: 10.3354/meps246183
Beninger P. G., St-Jean S. D. (1997). The role of mucus in particle processing by suspension-feeding marine bivalves: unifying principles. Mar. Biol. 129, 389–397. doi: 10.1007/s002270050179
Beninger P. G., St-Jean S., Poussart Y., Ward J. E. (1993). Gill function and mucocyte distribution in Placopecten magellanicus and Mytilus edulis (Mollusca: bivalvia): the role of mucus in particle transport. Mar. Ecol. Prog. Ser. 98, 275–282. doi: 10.3354/meps098275
Beninger P. G., Ward J. E., MacDonald B. A., Thompson R. J. (1992). Gill function and particle transport in Placopecten magellanicus (Mollusca: bivalvia) as revealed using video endoscopy. Mar. Biol. 114, 281–288. doi: 10.1007/BF00349531
Berman T., Townsend D. W., Elsayed S. Z., Trees C. C., Azov Y. (1984). Optical transparency, chlorophyll and primary productivity in the Eastern Mediterranean near the Israeli coast. Oceanol. Acta 7, 367–372.
Brickner I. (1985). Reproductive and settlement strategy of the boring bivalve lithophaga purpurea in living corals (Tel Aviv: Tel Aviv University).
Chesson J. (1983). The estimation and analysis of preference and its relationship to foraging models. Ecology 64, 1297–1304. doi: 10.2307/1937838
Cognie B., Barillé L., Massé G., Beninger P. G. (2003). Selection and processing of large suspended algae in the oyster Crassostrea gigas. Mar. Ecol. Prog. Ser. 250, 145–152. doi: 10.3354/meps250145
Cranford P. J., Emerson C. W., Hargrave B. T., Milligan T. G. (1998). In situ feeding and absorption responses of sea scallops Placopecten magellanicus (Gmelin) to storm-induced changes in the quantity and composition of the seston. J. Exp. Mar. Bio. Ecol. 219, 45–70. doi: 10.1016/S0022-0981(97)00174-3
Dadon-Pilosof A., Conley K. R., Jacobi Y., Haber M. (2017). Surface properties of SAR11 bacteria facilitate grazing avoidance. Nat. Microbiol. 2 (12), 1608–1615. doi: 10.1038/s41564-017-0030-5
Dadon-Pilosof A., Lombard F., Genin A. (2019). Prey taxonomy rather than size determines salp diets. Limnol. Oceanogr. 64, 1996–2010. doi: 10.1002/lno.11165
Dame R. F. (2013). “Bivalve filter feeders,” in Estuarine and coastal ecosystem processes. Ed. Dame R. F. (Springer Berlin Heidelberg), 565–569.
Diga R., Gilboa M., Moskovich R., Darmon N., Amit T., Belmaker J., et al. (2022). Invading bivalves replaced native Mediterranean bivalves, with little effect on the local benthic community. Biol. Invasions 25.5, 1–19. doi: 10.1007/s10530-022-02986-1
Dishon G., Dubinsky Z., Caras T., Rahav E., Bar-Zeev E., Tzubery Y., et al. (2012). Optical habitats of ultraphytoplankton groups in the gulf of eilat (Aqaba), northern red Sea. Int. J. Remote Sens. 33, 2683–2705. doi: 10.1080/01431161.2011.619209
Foladori P., Quaranta A., Ziglio G. (2008). Use of silica microspheres having refractive index similar to bacteria for conversion of flow cytometric forward light scatter into biovolume. Water Res. 42, 3757–3766. doi: 10.1016/j.watres.2008.06.026
Galil B. S. (2000). A sea under siege–alien species in the mediterranean. Biol. Invasions 2, 177–186. doi: 10.1023/A:1010057010476
Galil B. S., Gertman I., Gordon N., Herut B., Israel A., Lubinevsky H., et al. (2013). Biodiversity monitoring along the Israeli coast of the Mediterranean–activities and accumulated data. IOLR Rep. H 19.
Galil B. S., Zenetos A. (2002). “A sea change {{/amp]]mdash; exotics in the Eastern Mediterranean Sea,” in Invasive aquatic species of europe. distribution, impacts and management. Eds. Leppäkoski E., Gollasch S., Olenin S. (Dordrecht: Springer Netherlands), 325–336.
Gohar H. A. F., Soliman G. N. (1963). On three mytilid species boring in living corals. Publ. Mar. Biol. Sta. Al-Ghardaqa Red Sea 12, 65–98.
Hawkins A. J. S., Bayne B. L., Bougrier S., Héral M., Iglesias J. I. P., Navarro E., et al. (1998). Some general relationships in comparing the feeding physiology of suspension-feeding bivalve molluscs. J. Exp. Mar. Bio. Ecol. 219, 87–103. doi: 10.1016/S0022-0981(97)00176-7
Hawkins A. J. S., Smith R. F. M., Bayne B. L., Heral M. (1996). Novel observations underlying the fast growth of suspension-feeding shellfish in turbid environments: Mytilus edulis. Mar. Ecol. Prog. Ser. 131, 179–190. doi: 10.3354/meps131179
Hernroth B., Larsson A., Edebo L. (2000). Influence on uptake, distribution and elimination of Salmonella typhimurium in the blue mussel, Mytilus edulis, by the cell surface properties of the bacteria. J. Shellfish Res. 19 (1), 167–174.
Houlbrèque F., Ferrier-Pagès C. (2009). Heterotrophy in tropical scleractinian corals. Biol. Rev. Camb. Philos. Soc 84, 1–17. doi: 10.1111/j.1469-185X.2008.00058.x
Jackson G. A. (1980). Phytoplankton growth and zooplankton grazing in oligotrophic oceans. Nature 284, 439–441. doi: 10.1038/284439a0
Jacobi Y., Shenkar N., Ward J. E., Rosa M. (2021). Evasive plankton: size-independent particle capture by ascidians. Limnol. Oceanogr. 66, 1009–1020. doi: 10.1002/lno.11658
Jacobi Y., Yahel G., Shenkar N. (2018). Efficient filtration of micron and submicron particles by ascidians from oligotrophic waters. Limnol. Oceanogry. 63, S267–S279. doi: 10.1002/lno.10736
Johnson D. H. (1999). The insignificance of statistical significance testing. J. Wildl. Manage. 63, 763. doi: 10.2307/3802789
Jørgensen C. B. (1975). Comparative physiology of suspension feeding. Annu. Rev. Physiol. 37, 57–79. doi: 10.1146/annurev.ph.37.030175.000421
Jørgensen C. B. (1976). Comparative studies on the function of gills in suspension feeding bivalves, with special reference to effects of serotonin. Biol. Bull. 151, 331–343. doi: 10.2307/1540666
Jørgensen C. B. (1989). Water processing in ciliary feeders, with special reference to the bivalve filter pump. Comp. Biochem. Physiol. A Physiol. 94, 383–394. doi: 10.1016/0300-9629(89)90562-8
Jørgensen C. B., Kiorboe T., Mohlenberg F., Riisgård H. U. (1984). Ciliary and mucus-net filter feeding, with special reference to fluid mechanical characteristics. Mar. Ecol. Prog. Ser. 15, 283–292. doi: 10.3354/meps015283
Jørgensen C. B., Larsen P. S., Møhlenberg F., Riisgård H. U. (1988). The mussel pump: properties and modelling. Mar. Ecol. Prog. Ser., 205–216. doi: 10.3354/meps045205
Kach D. J., Ward J. E. (2008). The role of marine aggregates in the ingestion of picoplankton-size particles by suspension-feeding molluscs. Mar. Biol. 153, 797–805. doi: 10.1007/s00227-007-0852-4
Kleemann K. (1990). Coral associations, biocorrosion, and space competition in pedum spondyloideum (Gmelin) (Pectinacea, bivalvia). Mar. Ecol. 11, 77–94. doi: 10.1111/j.1439-0485.1990.tb00229.x
Klinker J., Reiss Z., Kropach C., Levanon I., Harpaz H., Shapiro Y. (1978). Nutrients and biomass distribution in the gulf of aqaba (Elat), red Sea. Mar. Biol. 45, 53–64. doi: 10.1007/BF00388977
Knap A. H., Michaels A., Close A. R., Ducklow H., Dickson A. G. (1996). “Protocols for the joint global ocean flux study (JGOFS) core measurements,” in JGOFS, reprint of the IOC manuals and guides no. 29 (UNESCO).
Krom M., Kress N., Berman-Frank I., Rahav E. (2014). “Past, present and future patterns in the nutrient chemistry of the Eastern Mediterranean,” in The Mediterranean Sea (Dordrecht: Springer Netherlands), 49–68.
Krom M. D., Kress N., Brenner S. (1991). Phosphorus limitation of primary productivity in the eastern Mediterranean Sea. Limnol. Oceanogr. 36 (3), 424–432. doi: 10.4319/lo.1991.36.3.0424
Kruschke J. K. (2010). What to believe: Bayesian methods for data analysis. Trends Cogn. Sci. 14, 293–300. doi: 10.1016/j.tics.2010.05.001
Langdon C. J., Newell R. I. E. (1990). Utilization of detritus and bacteria as food sources by two bivalve suspension-feeders, the oyster Crassostrea virginica and the mussel Geukensia demissa. Mar. Ecol. Prog. Ser. 58, 299–310. doi: 10.3354/meps058299
Lodola A., Nicolini L., Savini D., Deidun A. (2013). Range expansion and biometric features of Pinctada imbricata radiata (Bivalvia: pteriidae) around linosa island, central Mediterranean Sea (Italy). Ital. J. Zool. 80, 303–312. doi: 10.1080/11250003.2013.775363
Marquez A., Lodeiros C., Loor A., Revilla J., Da Costa F., Sonnenholzner S. (2019). Microalgae diet for juveniles of Spondylus limbatus. Aquac. Int. 27, 323–335. doi: 10.1007/s10499-018-0327-2
Mathieu-Resuge M., Schaal G., Kraffe E., Corvaisier R., Lebeau O., Lluch-Cota S. E., et al. (2019). Different particle sources in a bivalve species of a coastal lagoon: evidence from stable isotopes, fatty acids, and compound-specific stable isotopes. Mar. Biol. 166, 1–12. doi: 10.1007/s00227-019-3535-z
Mienis H. K., Galili E., Rapoport J. (1993). The spiny oyster, Spondylus spinosus, a well-established indo-pacific bivalve in the eastern Mediterranean off Israel (Mollusca, bivalvia, spondylidae). Zool. Middle East 9, 83–92.
Møhlenberg F., Riisgård H. U. (1978). Efficiency of particle retention in 13 species of suspension feeding bivalves. Ophelia 17, 239–246. doi: 10.1080/00785326.1978.10425487
Morganti T., Yahel G., Ribes M., Coma R. (2016). VacuSIP, an improved InEx method for In situ measurement of particulate and dissolved compounds processed by active suspension feeders. J. Vis. Exp. 2016, 1–14. doi: 10.3791/54221
Naumann M. S., Mayr C., Struck U., Wild C. (2010). Coral mucus stable isotope composition and labeling: experimental evidence for mucus uptake by epizoic acoelomorph worms. Mar. Biol. 157, 2521–2531. doi: 10.1007/s00227-010-1516-3
Naylor R. L., Hardy R. W., Buschmann A. H., Bush S. R., Cao L., Klinger D. H., et al. (2021). A 20-year retrospective review of global aquaculture. Nature 591, 551–563. doi: 10.1038/s41586-021-03308-6
Palmer R. E., Williams L. G. (1980). Effect of particle concentration on filtration efficiency of the bay scallop Argopecten irradians and the oyster Crassostrea virginica. Ophelia 19, 163–174. doi: 10.1080/00785326.1980.10425514
Pouvreau S., Jonquières G., Buestel D. (1999). Filtration by the pearl oyster, Pinctada margaritifera, under conditions of low seston load and small particle size in a tropical lagoon habitat. Aquaculture 176, 295–314. doi: 10.1016/S0044-8486(99)00102-7
Qiao L., Chang Z., Li J., Li T. (2022). Selective feeding of three bivalve species on the phytoplankton community in a marine pond revealed by high-throughput sequencing. Sci. Rep. 12, 1–13. doi: 10.1038/s41598-022-08832-7
Riisgård H. U. (1988). Efficiency of particle retention and filtration rate in 6 species of northeast American bivalves. Mar. Ecol. Prog. Ser. 45 (3), 217–223. doi: 10.3354/meps045217
Riisgård H. U. (2001). The stony road to reliable filtration rate measurements in bivalves: a reply. Mar. Ecol. Prog. Ser. 215, 307–310. doi: 10.3354/meps215307
Riisgård (2002). Methods of ciliary filter feeding in adult Phoronis muelleri (phylum phoronida) and in its free-swimming actinotroch larva. Mar. Biol. 141, 75–87. doi: 10.1007/s00227-002-0802-0
Riisgård H. U., Kittner C., Seerup D. F. (2003). Regulation of opening state and filtration rate in filter-feeding bivalves (Cardium edule, mytilus edulis, mya arenaria) in response to low algal concentration. J. Exp. Mar. Bio. Ecol. 284, 105–127. doi: 10.1016/S0022-0981(02)00496-3
Riisgård H. U., Larsen P. S. (2010). Particle capture mechanisms in suspension-feeding invertebrates. Mar. Ecol. Prog. Ser. 418, 255–293. doi: 10.3354/meps08755
Riisgård H. U., Larsen P. S., Nielsen N. F. (1996). Particle capture in the mussel Mytilus edulis: the role of latero-frontal cirri. Mar. Biol. 127, 259–266. doi: 10.1007/BF00942111
Rilov G. (2009). Biological invasions in marine ecosystems: ecological, management, and geographic perspectives. Eds. Rilov G., Crooks J. A. (Berlin, Germany: Springer).
Rilov G., Benayahu Y., Gasith A. (2004). Prolonged lag in population outbreak of an invasive mussel: a shifting-habitat model. Biol. Invasions 6, 347–364. doi: 10.1023/B:BINV.0000034614.07427.96
Rosa M., Evan Ward J., Shumway S. E. (2018). Selective capture and ingestion of particles by suspension-feeding bivalve molluscs: a review. J. Shellfish Res. 37, 727–746. doi: 10.2983/035.037.0405
Rosa M., Ward J. E., Frink A., Shumway S. E. (2017). Effects of surface properties on particle capture by two species of suspension-feeding bivalve molluscs. Am. Malacol. Bull. 35, 181–188. doi: 10.4003/006.035.0212
Rosa M., Ward J. E., Shumway S. E., Wikfors G. H. (2013). Effects of particle surface properties on feeding selectivity in the eastern oyster Crassostrea virginica and the blue mussel Mytilus edulis. J. Exp. Mar. Bio. Ecol. 446, 320–327. doi: 10.1016/j.jembe.2013.05.011
Sarà G. (2006). Hydrodynamic effects on the origin and quality of organic matter for bivalves: an integrated isotopic, biochemical and transplant study. Mar. Ecol. Prog. Ser. 328, 65–73. doi: 10.3354/meps328065
Sarà G., Romano C., Caruso M., Mazzola A. (2000). The new lessepsian entry Brachidontes pharaonis (Fischer p. 1870) (Bivalvia, mytilidae) in the western Mediterranean: a physiological analysis under varying natural conditions. J. Shellfish Res. 19 (2), 967–977.
Sarà G., Romano C., Widdows J., Staff F. J. (2008). Effect of salinity and temperature on feeding physiology and scope for growth of an invasive species (Brachidontes pharaonis - MOLLUSCA: BIVALVIA) within the Mediterranean Sea. J. Exp. Mar. Bio. Ecol. 363, 130–136. doi: 10.1016/j.jembe.2008.06.030
Sarà G., Vizzini S., Mazzola A. (2003). Sources of carbon and dietary habits of new lessepsian entry Brachidontes pharaonis (Bivalvia, mytilidae) in the western Mediterranean. Mar. Biol. 143, 713–722. doi: 10.1007/s00227-003-1118-4
Shabtay A., Rilov G., Benayahu Y. (2015). The indo-pacific oyster spondylus spinosus schreibers 1793 in the Eastern Mediterranean Sea: reproductive features. Molluscan Res. 35, 206–212. doi: 10.1080/13235818.2015.1007534
Shafir A., Loya A. (1983). “Consumption and assimilation of coral mucus by the burrowing mussel Lithophaga lessepsiana,” in Proc Int Conf Mar Sci Red Sea. 135–140.
Shaked Y., Genin A. (2018). Israel National monitoring program in the northern gulf of Eilat/Aqaba–scientific report for the year 2017.
Sieburth J. M., Smetacek V., Lenz J. (1978). Pelagic ecosystem structure: heterotrophic compartments of the plankton and their relationship to plankton size fractions 1. Limnol. Oceanogr. 23, 1256–1263. doi: 10.4319/lo.1978.23.6.1256
Siegel D. A., Dickey T. D., Washburn L., Hamilton M. K., Mitchell B. G. (1989). Optical determination of particulate abundance and production variations in the oligotrophic ocean. Deep Sea Res. A 36, 211–222. doi: 10.1016/0198-0149(89)90134-9
Signor P. W., Vermeij G. J. (1994). The plankton and the benthos: origins and early history of an evolving relationship. Paleobiology 20, 297–319. doi: 10.1017/S0094837300012793
Silverman H., Achberger E. C., Lynn J. W. (1995). Filtration and utilization of laboratory-cultured bacteria by Dreissena polymorpha, Corbicula fluminea, and Carunculina texasensis. Biol. Bull. 189 (3), 308–319. doi: 10.2307/1542148
Silverman H., Lynn J. W., Beninger P. G., Dietz T. H. (1999). The role of latero-frontal cirri in particle capture by the gills of Mytilus edulis. Biol. Bull. 197, 368–376. doi: 10.2307/1542791
Smaal A. C., Ferreira J. G., Grant J., Petersen J. K., Strand Ø. (2019). Goods and services of marine bivalves (Springer Nature).
Sokolova I. M., Frederich M., Bagwe R., Lannig G., Sukhotin A. A. (2012). Energy homeostasis as an integrative tool for assessing limits of environmental stress tolerance in aquatic invertebrates. Mar. Environ. Res. 79, 1–15. doi: 10.1016/j.marenvres.2012.04.003
Sonier R., Filgueira R., Guyondet T., Tremblay R., Olivier F., Meziane T., et al. (2016). Picophytoplankton contribution to Mytilus edulis growth in an intensive culture environment. Mar. Biol. 163 (4), 1–15. doi: 10.1007/s00227-016-2845-7
Vahl O. (1972). Particle retention and relation between water transport and oxygen uptake in Chlamys opercularis (Bivalvia). Ophelia 10, 67–74. doi: 10.1080/00785326.1972.10430104
Velasco L. A., Navarro J. M. (2005). Feeding physiology of two bivalves under laboratory and field conditions in response to variable food concentrations. Mar. Ecol. Prog. Ser. 291, 115–124. doi: 10.3354/meps291115
Ward J. E., Kach D. J. (2009). Marine aggregates facilitate ingestion of nanoparticles by suspension-feeding bivalves. Mar. Environ. Res. 68, 137–142. doi: 10.1016/j.marenvres.2009.05.002
Ward J. E., Sanford L. P., Newell R. I. E., MacDonald B. A. (1998). A new explanation of particle capture in suspension- feeding bivalve molluscs. Limnol. Oceanogr. 43, 741–752. doi: 10.4319/lo.1998.43.5.0741
Ward J. E., Shumway S. E. (2004). Separating the grain from the chaff: particle selection in suspension-and deposit-feeding bivalves. J. Exp. Mar. Bio. Ecol. 300, 83–130. doi: 10.1016/j.jembe.2004.03.002
Yahel R., Frid O. (2018). Marine BioBlitz – marine BioBlitz-biological survey of the israelis MPAs in the Mediterranean Sea-spring and fall 2015 (Jerusalem: Israel Nature and Parks Authority).
Yahel G., Marie D., Beninger P. G., Eckstein S., Genin A. (2009). In situ evidence for pre-capture qualitative selection in the tropical bivalve Lithophaga simplex. Aquat. Biol. 6, 235–246. doi: 10.3354/ab00131
Yahel G., Marie D., Genin A. (2005). InEx-a direct in situ method to measure filtration rates, nutrition, and metabolism of active suspension feeders. Limnol. Oceanogr. Methods 3, 46–58. doi: 10.4319/lom.2005.3.46
Keywords: bivalves feeding, coral-boring bivalves, picoplankton, particle capture, in situ, oligotrophic
Citation: Amit T, Moskovich R, Jacobi Y, Shumway SE, Ward JE, Beninger P, Yahel G and Loya Y (2023) Feeding on the smallest cells: an in situ study of picoplankton capture by bivalve molluscs from oligotrophic waters. Front. Mar. Sci. 10:1184773. doi: 10.3389/fmars.2023.1184773
Received: 12 March 2023; Accepted: 15 May 2023;
Published: 30 May 2023.
Edited by:
Caterina Longo, University of Bari Aldo Moro, ItalyReviewed by:
Pere Ferriol, University of the Balearic Islands, SpainJihong Zhang, Chinese Academy of Fishery Sciences (CAFS), China
Copyright © 2023 Amit, Moskovich, Jacobi, Shumway, Ward, Beninger, Yahel and Loya. This is an open-access article distributed under the terms of the Creative Commons Attribution License (CC BY). The use, distribution or reproduction in other forums is permitted, provided the original author(s) and the copyright owner(s) are credited and that the original publication in this journal is cited, in accordance with accepted academic practice. No use, distribution or reproduction is permitted which does not comply with these terms.
*Correspondence: Tal Amit, talamit83@gmail.com