Reactive gliosis in traumatic brain injury: a comprehensive review
- 1Department of Neuroscience, Second Faculty of Medicine, Charles University, Prague, Czechia
- 2Department of Cellular Neurophysiology, Institute of Experimental Medicine of the Czech Academy of Sciences, Prague, Czechia
Traumatic brain injury (TBI) is one of the most common pathological conditions impacting the central nervous system (CNS). A neurological deficit associated with TBI results from a complex of pathogenetic mechanisms including glutamate excitotoxicity, inflammation, demyelination, programmed cell death, or the development of edema. The critical components contributing to CNS response, damage control, and regeneration after TBI are glial cells–in reaction to tissue damage, their activation, hypertrophy, and proliferation occur, followed by the formation of a glial scar. The glial scar creates a barrier in damaged tissue and helps protect the CNS in the acute phase post-injury. However, this process prevents complete tissue recovery in the late/chronic phase by producing permanent scarring, which significantly impacts brain function. Various glial cell types participate in the scar formation, but this process is mostly attributed to reactive astrocytes and microglia, which play important roles in several brain pathologies. Novel technologies including whole-genome transcriptomic and epigenomic analyses, and unbiased proteomics, show that both astrocytes and microglia represent groups of heterogenic cell subpopulations with different genomic and functional characteristics, that are responsible for their role in neurodegeneration, neuroprotection and regeneration. Depending on the representation of distinct glia subpopulations, the tissue damage as well as the regenerative processes or delayed neurodegeneration after TBI may thus differ in nearby or remote areas or in different brain structures. This review summarizes TBI as a complex process, where the resultant effect is severity-, region- and time-dependent and determined by the model of the CNS injury and the distance of the explored area from the lesion site. Here, we also discuss findings concerning intercellular signaling, long-term impacts of TBI and the possibilities of novel therapeutical approaches. We believe that a comprehensive study with an emphasis on glial cells, involved in tissue post-injury processes, may be helpful for further research of TBI and be the decisive factor when choosing a TBI model.
1 Introduction
Traumatic brain injury (TBI) is one of the most common types of brain injury, with major medical and socio-economic problems (Hyder et al., 2007; Rubiano et al., 2015). It is approximated that TBI annually afflicts a range of 54 to 60 million individuals, necessitating hospitalization or culminating in mortality. Worldwide, TBI accounted for 27.16 million new cases (with 95% uncertainty intervals of 23.36 to 31.42 million), 48.99 million prevalent cases (46.84 to 51.32 million), and 7.08 (5.00 to 9.59 million) million years lived with disability (YLDs) in 2019 (Guan et al., 2023). On a global scale, the age-standardized incidence rates of TBI have demonstrated a significant decrease since 1990. This positive trend suggests potential benefits arising from international initiatives aimed at lowering TBI rates. Notably, substantial efforts have been directed toward enhancing road safety and mitigating road-related injuries, a prominent contributor to TBI globally. Among the diverse injury categories, cerebral injuries exhibit a heightened propensity to resulting in either death or sustained disabilities (Hyder et al., 2007). The primary factors contributing to TBI vary by age, socio-economic conditions and geographical locations. Low and medium-income countries report an approximately threefold higher proportional incidence of TBI compared to high-income countries. The Southeast Asian and Western Pacific regions bear the highest overall burden in this regard (Dewan et al., 2018). According to studies, falls have been identified as the predominant etiological factor for TBI (74%), followed by road injuries (19%, mainly Africa and Southeast Asia), violence (2%, South America, Caribbean and Sub Saharan Africa), and sports- and work-related incidents (Iaccarino et al., 2018). Over 25% of individuals with long-term mild traumatic brain injury (mTBI) consequences struggle to return to work even a year post-injury (Langlois et al., 2006). Chronic mTBI represents a formidable health challenge characterized by lifelong disabilities and enduring consequences, significantly diminishing the affected individuals’ quality of life (Seabury et al., 2018). The economic ramifications are noteworthy; the estimated overall healthcare cost attributable to non-fatal TBI in 2016 was, with rehabilitation costs per patient reaching $36.000, contributing to an annual national expenditure of nearly $40.6 billion (Miller et al., 2021). Despite these profound implications, the molecular mechanisms underpinning chronic mTBI symptoms remain elusive within current scientific understanding.
Traumatic brain injury is a complex process, in which the primary injury followed by the secondary injury evokes pathological structural changes combined with functional deficits (Galgano et al., 2017). The term primary injury refers to an initial insult caused by mechanical forces, that depending on the intensity/severity and type of injury, may result in contusion or penetration of the brain and/or blood-brain barrier (BBB) disruption. The primary injury can evoke a focal or diffuse type of tissue damage. Diffuse injury occurs with a higher frequency than a focal one, however, a combination of both types is common in patients (Skandsen et al., 2010). The secondary tissue damage occurs over a period of time, from minutes up to several days after the primary injury, and is caused by cellular reactions, activation of metabolic pathways associated with neuroinflammation, edema, hypoxia, or tissue atrophy (Ng and Lee, 2019). The damage and the extent of the injury caused by TBI are determined by the severity of the injury, which can be assessed by various methods, including the Glasgow Coma Score (GCS). The GCS scale evaluates three parameters (eye, verbal, and motor responses) and based on the scores, the injury is classified as mild (GCS 13–15), moderate (GCS 9–12), or severe (GCS 3–8) (Jain and Iverson, 2022). The majority of reported TBI in patients is of mild level (Leo and McCrea, 2016), but numbers of cases may be higher as numerous patients with suspected mTBI do not seek medical help after an accident. Additionally, the period following TBI can be divided into three phases: acute (24 h post-TBI), subacute (1 day–3 weeks post-TBI), and chronic phase (more than 3 weeks post-TBI). However, these time periods are only general and may vary from case to case as they are affected by various factors, such as age, injury type and location (Lund et al., 2016; Toshkezi et al., 2018).
Secondary brain injury comprises a complex series of cascading events within the brain. These events include the release of pro-inflammatory cytokines, chemokines, and signaling molecules, which create a pro-inflammatory microenvironment. This environment exacerbates neuronal excitotoxicity and oxidative stress, contributing significantly to the development of secondary brain damage (Ng and Lee, 2019). Glial cells, representing the most abundant brain cells, play a prominent role in these events, as well as in synaptic pruning, synaptic plasticity alterations, and the long-term functional outcomes of the injured brain, emphasizing their central position in the pathophysiology of secondary brain injury (Mira et al., 2021).
In response to tissue trauma, glia cells undergo both morphological and functional changes characterized by the term reactive gliosis, which usually refers to astrocytes and microglia (Buffo et al., 2008; Gao et al., 2013; Pekny and Pekna, 2016). Reactive glia, as part of the lesion, are involved in neuroinflammation and edema to varying degrees, create the glial scar and produce extracellular matrix (ECM) molecules, such as chondroitin sulfate proteoglycans (CSPGs) that contribute to glial scar formation and stabilize it (Asher et al., 2001; Dyck and Karimi-Abdolrezaee, 2015). Moreover, reactive glia produce a large spectrum of proteins, including growth factors and cytokines that are crucial for intercellular cooperation as well as for protective mechanisms and regeneration of damaged tissue (Ziebell and Morganti-Kossmann, 2010).
In the healthy brain, astrocytes are the main effector cells responsible for the optimal environment for neuronal survival and functions: they maintain ion/pH/water homeostasis and BBB integrity, modulate synaptic activity, ensure the neurotransmitter clearance from the extracellular space and are involved in neuronal metabolism, by providing energy substrates as well as by metabolite removal (Kim et al., 2019). During TBI, besides being a key component forming glial scar, reactive astrocytes produce pro-inflammatory cytokines and chemokines (Gyoneva and Ransohoff, 2015; Michinaga and Koyama, 2021). Changes in the expression and localization of various astrocytic proteins forming ion channels and transporters, such as aquaporin-4 (AQP4) or glutamate transporters EAAT1/GLT-1 and EAAT2/GLAST, also contribute to edema development and excitotoxicity (van Landeghem et al., 2006; Beschorner et al., 2007; Kapoor et al., 2013). Unlike astrocytes, the functions of microglia are much more narrowly focused and their main function under physiological conditions is immune surveillance and the phagocytosis of apoptotic debris. However, it has also been demonstrated that microglia can be involved in synapse formation and maintaining synaptic homeostasis, as well as in the production of a variety of growth factors modulating neuronal activity (Borst et al., 2021). In the injured brain, microglia are the key components of neuroinflammation, and together with astrocytes they contribute to scar formation (Wake et al., 2009; Miyamoto et al., 2013). Oligodendrocytes are myelinating cells providing support and insulating myelin sheaths to axons. Pathologies such as TBI accompanied by oxidative stress or excitotoxicity have a detrimental effect on oligodendrocytes, resulting in their apoptosis and demyelination, which can significantly affect the resulting post-TBI functional outcome (Dent et al., 2015). There is increasing evidence of the important role of oligodendrocyte progenitor cells (OPCs), also known as polydendrocytes or NG2-glia, in brain pathologies, including TBI (Dimou and Gallo, 2015; Akay et al., 2021). NG2-glia have a large capacity to proliferate and differentiate into other cellular types, mostly in myelinating oligodendrocytes under physiological conditions (Zhu et al., 2008; Dimou and Gallo, 2015). In the injured brain, NG2-glia become part of the lesion and glial scar, contribute to wound closure, regulate neuroinflammation, and besides oligodendrocytes, NG2-glia can differentiate into other cell types, especially reactive astrocytes which seem to be injury type-dependent (Honsa et al., 2016; Hackett et al., 2018).
Traumatic brain injury research articles are mostly aimed at astrocytes and microglia, while NG2-glia and oligodendrocytes are usually neglected. In this review, we focus on the roles of these four types of glial cells in secondary injury pathologies based on observations in the different TBI models and factors that may impact the outcomes and should be considered when designing experiments.
2 Experimental models of traumatic brain injury
Traumatic brain injury is a highly variable and complex process, and there is currently no animal model which would be able to completely reproduce all the changes that occur after TBI. To date, a number of TBI models have been developed that differ in the type of the induced injury (focal vs. diffuse; impacted vs. non-impacted) as well as in the severity of the tissue damage. Here, we list a brief overview of the most known models: Weight drop injury (WD), Controlled cortical impact (CCI), Fluid percussion injury (FPI), Blast injury (BI), Penetrating ballistic-like brain injury (PBBI) and Close-head impact model of engineered rotational acceleration (CHIMERA) [for comprehensive reviews see (Xiong et al., 2013; McDonald et al., 2021)]. With regards to the necessary equipment, the WD model is the simplest to perform, while the others require sophisticated devices. Rodents are the most used animals for TBI experiments, but animals such as zebrafish (Hentig et al., 2021) and Drosophila (Katzenberger et al., 2013) are used as well. The CCI and WD models can be produced as an open-head or closed-head injury (CHI), FPI and PBBI are open-head type of models, while BI and CHIMERA are CHI models. Open head models require a craniotomy, and the impact is directed to the surface of the dura. CHI is a type of TBI, where the force impacts on the intact skull (Deshetty and Periyasamy, 2023).
2.1 Weight drop (WD)
This model is performed by a free fall of guided weight to an exposed skull (depending on the model, with or without craniotomy) (Figure 1). There are 3 basic types of this model: 1. Feeney’s model exposes the dura, and the weight impact results in cortical contusion followed by the development of a necrotic cavity (Feeney et al., 1981). 2. Shohami’s model is a CHI model, where weight impacts an unprotected skull. Due to this model, edema occurs in the traumatized hemisphere with the addition of hemorrhagic lesions which in turn develop into necrosis (Shapira et al., 1988). 3. The Marmarou’s model uses a metal disc to prevent skull fractions. This model mimics TBI induced by falls and motor vehicle accidents and evokes a diffuse injury without lesion development (Marmarou et al., 1994). The severity of the WD model can be adjusted by changes of the weight mass and/or height of the drop of the weight (Ma et al., 2019). The WD model replicates human TBI fairly well, and the WD device is simple and affordable in comparison to other models. However, complications with skull fractures, high mortality, and variability in observed injuries may emerge (Zhang et al., 2014).
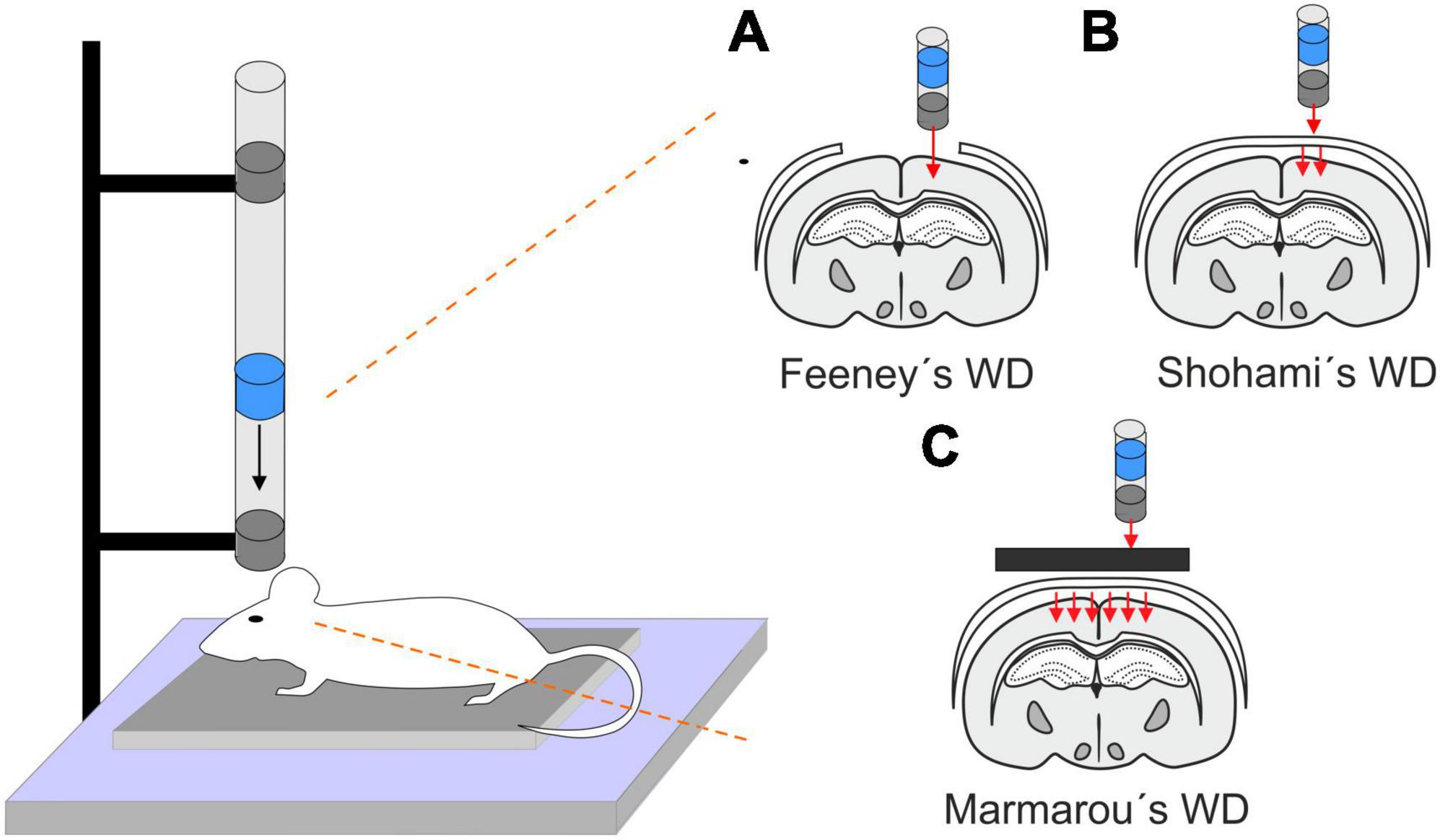
Figure 1. Models of traumatic brain injury - Weight drop (WD). WD models use free fall of guided weight in different modifications. (A) In Feeney’s WD model, the weight drops on the exposed dura. (B) In Shohami’s WD model, the weight impacts an unprotected scull. (C) In Marmarou’s WD model, the weight is dropped on a metal disc or other scull protection and simulates a diffuse concussive head injury for example in football players or car accidents.
2.2 Controlled cortical impact (CCI)
This model uses a controlled piston (electromagnetic or pneumatic control) which delivers an impact to the exposed dura (Osier and Dixon, 2016; Figure 2A). This type of model evokes a focal brain injury resulting in cortical tissue loss, axonal injury, BBB disruption and development of edema. Moreover, this model was adapted to be used for close head injury, CCI-CHI (Romine et al., 2014; Osier and Dixon, 2016). The severity of the resulting injury can be adjusted by controlling the depth impact and the velocity of the piston (Ma et al., 2019).
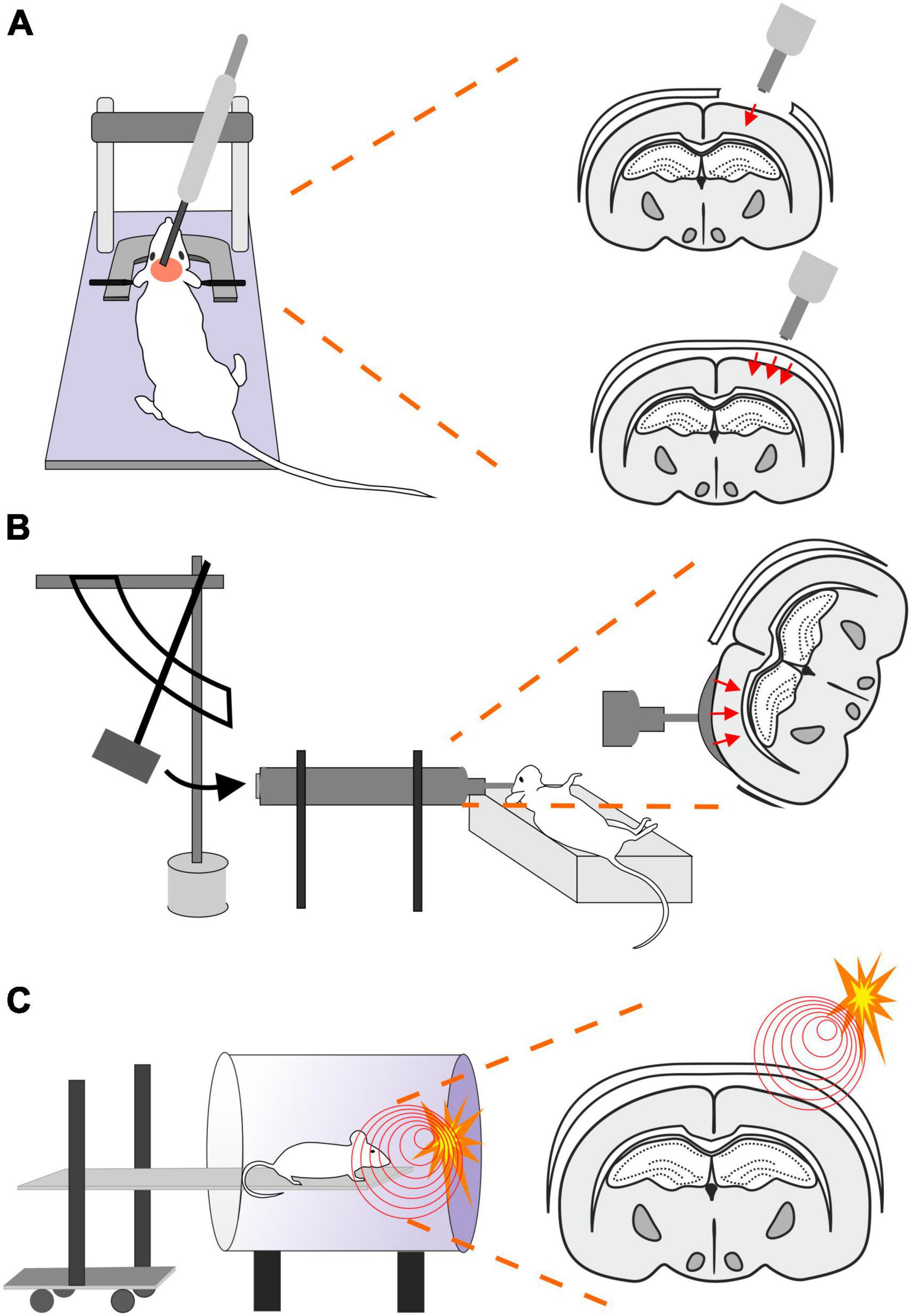
Figure 2. Models of traumatic brain injury–Controlled cortical impact (CCI), Fluid percussion injury (FPI) and Blast injury (BI). (A) In CCI model, pneumatic- or an electromagnetic-controlled piston impact the exposed dura. The severity of the evoked focal TBI depends on the depth of the impact and the velocity of the piston impacting either the exposed dura (frequently used as a model of focal/open head injury; upper scheme) or unprotected skull (modified CCI to mimick diffuse/closed head injury; lower scheme). Moreover, the TBI severity is also affected by the material of the pad (foam vs. solid) and impactor tip (rubber vs. solid) or by the presence or absence of head fixation. (B) Brain injury in FPI model is evoked by a fluid pulse transferred to exposed dura by the impact of a pendulum on a piston filled with fluid. This mode mimics brain contusion without scull fraction and its outcome depends on the localization of the craniotomy and the angle of the pendulum. (C) In the BI model, the animal is placed on a movable platform in a shock tube capable of controlling overpressure waves evoked by a controlled detonation. The model simulates diffuse mTBI often seen in military personnel.
The advantages of this model are the low mortality rate, no risk of rebound injury, and result reproducibility. However, it also depends on the impactor diameter and whether the simple CCI or adapted CCI-CHI is used (Zhang et al., 2014; Ma et al., 2019).
2.3 Fluid percussion injury (FPI)
The FPI model uses a fluid pulse delivered to the dura by the impact of a pendulum on a piston filled with fluid (Ma et al., 2019; Figure 2B). Based on the placement, this technique can be used for lateral FPI (a combination of focal and diffuse injury) or midline FPI (diffuse injury). FPI simulates brain contusion without skull fracture. Localization of the craniotomy is an important factor when using this model. Floyd et al. (2002) tested 4 groups with rostral, caudal, medial, and lateral craniotomy. The caudal group exhibited a higher mortality rate than other groups after FPI, and the rostral group showed the lowest level of cellular loss in the hippocampal area. The authors also showed differences in the levels of reactive astrogliosis in a brain area-dependent manner, whereas the rostral group showed less immunoreactivity for astrocytic marker glial fibrillary acidic protein (GFAP) than the rest of the groups. However, it should be noted that fewer animals per group were used for the GFAP expression tests than for the rest of the aforementioned examinations (Floyd et al., 2002). The FPI model fairly accurately mimics the glial reaction to TBI, as the studies using this model reported hypertrophy of astrocytes with different orientation, and a decreased number of their processes or structural changes of microglia (rod-like morphology) (Ziebell et al., 2012; Robinson et al., 2016). The FPI model can be adjusted by setting the angle of the pendulum higher or lower (Eakin et al., 2015). This model results in direct brain trauma without the necessity of skull protection, and the mortality rate is relatively low. The disadvantage of this model is the necessity of fluid percussion system monitoring during the procedure, since factors such as residual air in the system can cause variability or added injuries (Zhang et al., 2014).
2.4 Blast injury (BI)
Blast-induced traumatic brain injury (bTBI) is caused by exposure to explosive devices. This type of injury is often called “invisible injury” because there is no evidence of an external injury (Lucci, 2006). This form of neurotrauma represents the most prevalent type of mTBI in the context of military personnel. Blast waves cause significant oscillating acceleration-deceleration cycles on the head, which is known as the “bobblehead effect” (Rosenfeld et al., 2013). The detonation of an explosive device generates a supersonic pressure gradient, caused by a primary blast wave. This wave consists of a high positive pressure followed by a prolonged negative pressure phase, forming a Friedlander curve. Primary bTBI is characterized by internal injuries that are challenging to detect and assess for severity. Strong blasts can cause acute injury or death, while exposure to milder forces may result in delayed or subclinical neuropathological changes. To simulate bTBI, a shock tube capable of controlling overpressure waves is utilized to mimick real-life free-field explosions, resembling the Friedlander-type waveform (Huber et al., 2013; Campos-Pires et al., 2018; Figure 2C).
2.5 Penetrating ballistic-like brain injury (PBBI)
Penetrating ballistic-like brain injury (PBBI) results from high-energy projectiles, creating a substantial temporary cavity in the brain (Williams et al., 2005, 2006; Figure 3A). The outcome is closely linked to the projectile’s anatomical path and energy transfer (Williams et al., 2005). Using this model in rats results in cognitive impairment, white and gray matter damage, brain swelling, seizures, cortical spreading depression, and neuroinflammation (Williams et al., 2007). Studies using new rodent PBBI models, including a non-fatal model which uses a modified air rifle for low-velocity PBBI or another PBBI model simulating the effect of high-energy projectiles, reveal immediate and subacute changes such as increased intracranial pressure, BBB permeability, brain edema formation, and persistent motor and cognitive deficits (Plantman et al., 2012). The unique aspect of extensive intracerebral hemorrhage makes PBBI a valuable model for studying moderate-to-severe brain trauma as well as for assessing the effect of therapeutic interventions (Williams et al., 2006; Chen et al., 2009; Shear et al., 2009).
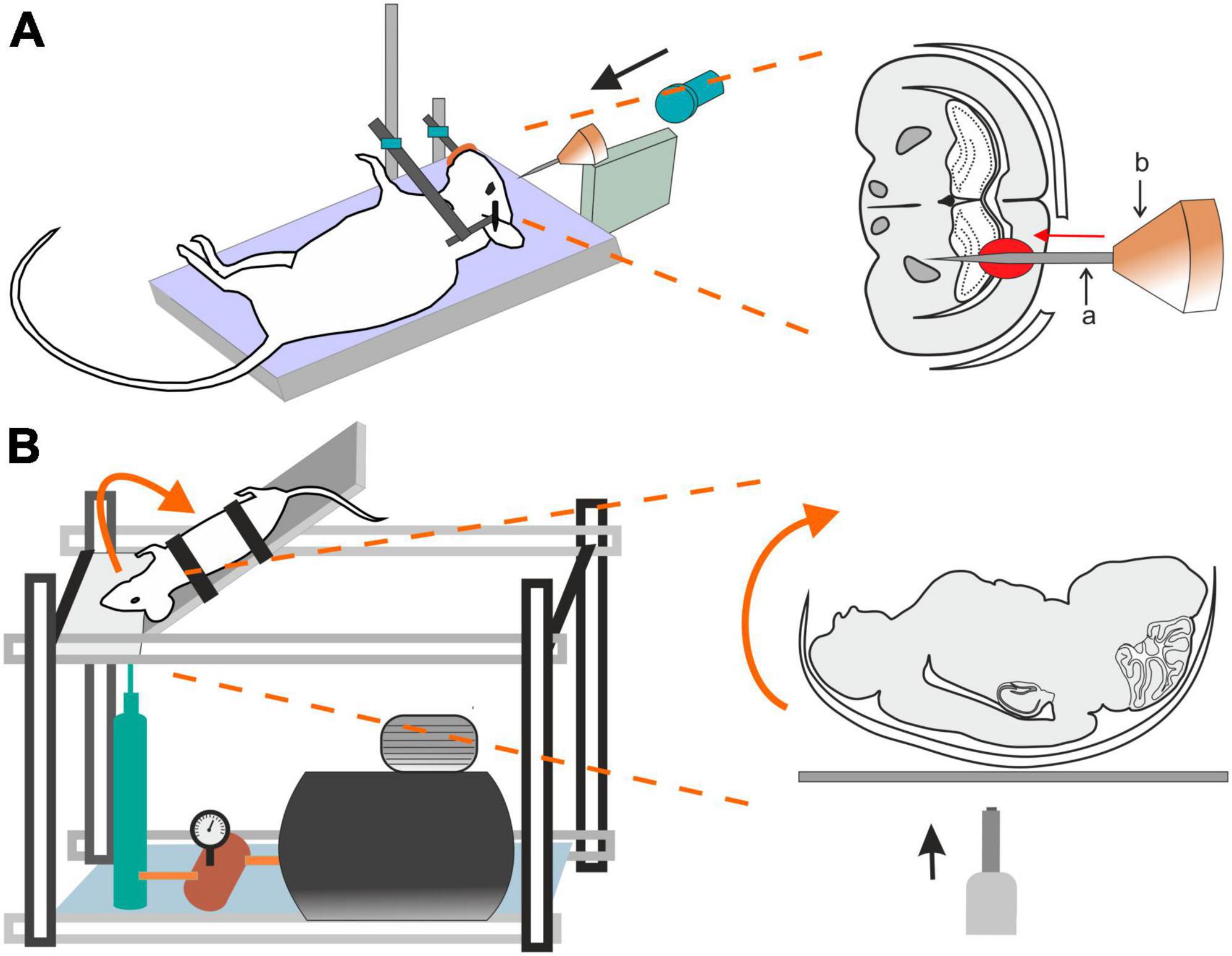
Figure 3. Models of traumatic brain injury–Penetrating ballistic-like brain injury (PBBI) and the Closed-head impact model of engineered rotational acceleration (CHIMERA). (A) In the PBBI model, a modified air-rifle is utilized to accelerate a projectile (led pellet) into a conic impactor probe (a) placed in an impactor holder (b) against the animal head which is fixed by stereotactic apparatus and a nose clip. Probe penetration results in the creation of temporary brain cavity, mimicking the damage after a gun shot. The severity of the injury can be modified by adjusting the air-rifle pressure. (B) In the device utilized for a mouse CHIMERA, a piston, driven by a high-pressure pulse, impacts the plate which the animal’s head is freely resting on, while the animal body is fixed in a supine position. The impact results in a sagittal plane motion of the animal head.
2.6 Closed-head impact model of engineered rotational acceleration (CHIMERA)
This model is highlighted for its relevance in studying the impact of the acceleration effects associated with major trauma, including falls, vehicular accidents, and sports-related collisions (Namjoshi et al., 2014) and is particularly important for modeling axonal and white matter injury (Bashir et al., 2020; Krieg et al., 2023). CHIMERA’s commercial availability is emphasized, providing researchers with the means to standardize injury parameters and ensure consistent replication across various laboratories. Specifically, the mouse-oriented CHIMERA device exhibits compressive contact and inertial forces, along with changes in velocity and angular velocity akin to values observed in professional football and boxing (Namjoshi et al., 2014).
The CHIMERA TBI model utilizes the high-pressure impact from a metal piston on the platform under the animal’s head (Figure 3B). While the animal head rests freely, its body is secured in a supine position with straps, aided by crosshairs for precise head alignment. Compressed air, regulated by a valve, propels the piston, and its velocity is measured by infrared devices. A pressure gauge regulates impact intensity. Upon device activation, the ascending platform initiates a forward swing of the animal head that touched the sternum before returning to the original position on the platform (McNamara et al., 2020). All CHIMERA models involve animals in a supine position, causing sagittal plane motion. CHIMERA device reconfiguration enables a comparison of sagittal and horizontal impacts, shedding light on varying functional and morphological outcomes associated with different injury planes (Browne et al., 2011; Mychasiuk et al., 2016).
2.7 Repeated mild traumatic brain injury
The protocols of WD, CCI, FPI, and BI can be adapted to produce only mTBI by adjustment of the velocity, depth of duration of a traumatic pulse/impact. To simulate the head trauma often seen in contact sports, models of repetitive mild traumatic brain injuries (rmTBIs) have been introduced, where each single injury is below the concussion threshold, but their effects add up and may result in prolonged and/or delayed tissue damage and neurological deficit. The parameters of experimental protocols and observed alteration in biochemical/neuropathological analysis as well as in neurological outcome in different studies is thoroughly described in a systematic review of Hiskens et al. (2019).
2.8 Pediatric traumatic brain injury models
Traumatic brain injury is a significant global issue in infants and children. Head injuries in children can lead to a variety of traumatic injuries to the scalp, skull, and brain, mirroring those in adults. The necessity to study developmental TBI and its long-term consequences in appropriate models arose from significant distinctions in blood flow, metabolic rate, neurotransmitter activity, degree of myelination, ability to tolerate oxidative stress, and/or biomechanical scull properties between adult and the pediatric population (Margulies and Thibault, 2000; Morrison et al., 2013; Araki et al., 2017). For simulation of pediatric TBI, already established modified models such as WD, FPI, CCI, CCI-CHI, CHIMERA, and rmTBI models are employed as well as specific models for abusive head trauma (AHT), commonly known as shaken baby syndrome, which is the predominant form of TBI in infants <1 year (Bonnier et al., 2004). Experimental animals include rodents aged 11–21 days, covering responses from a term infant (aged 7–11 days) to a toddler (aged 17–21 days), piglets (especially in FPI and CHIMERA models) and lambs (shake injury models). Further insights into this topic are provided by comprehensive reviews focusing on the considerable challenges in the field and highlighting essential models that investigate the unique injury mechanisms related to pediatric TBI (Araki et al., 2017; Kochanek et al., 2017; Nwafor et al., 2022).
3 The response of glial cells to traumatic brain injury
Both human brain trauma as well as its experimental models induce a typical response of nervous tissue that is based predominantly on glia reaction and include:
3.1 Edema
Traumatic brain injury is typically accompanied by cerebral edema, which is characterized by an increase and retention of CNS water content, contributing to elevated intracranial pressure. Two types of edema can be present post-TBI: vasogenic and cellular/cytotoxic edema. The vasogenic edema accompanies the compromised BBB integrity after TBI and it is characterized by an extracellular accumulation of fluid. The vasogenic type of edema prevails in the first days post-TBI; while the disrupted BBB gradually closes, cytotoxic edema with a slower onset follows (Donkin and Vink, 2010; Figure 4). Cytotoxic edema, the most common type of edema found in TBI patients (Marmarou et al., 2006), results in cellular swelling (including astrocytes), which can eventually result in apoptosis. However, this type of edema does not contribute to brain swelling or an increase in intracranial pressure.
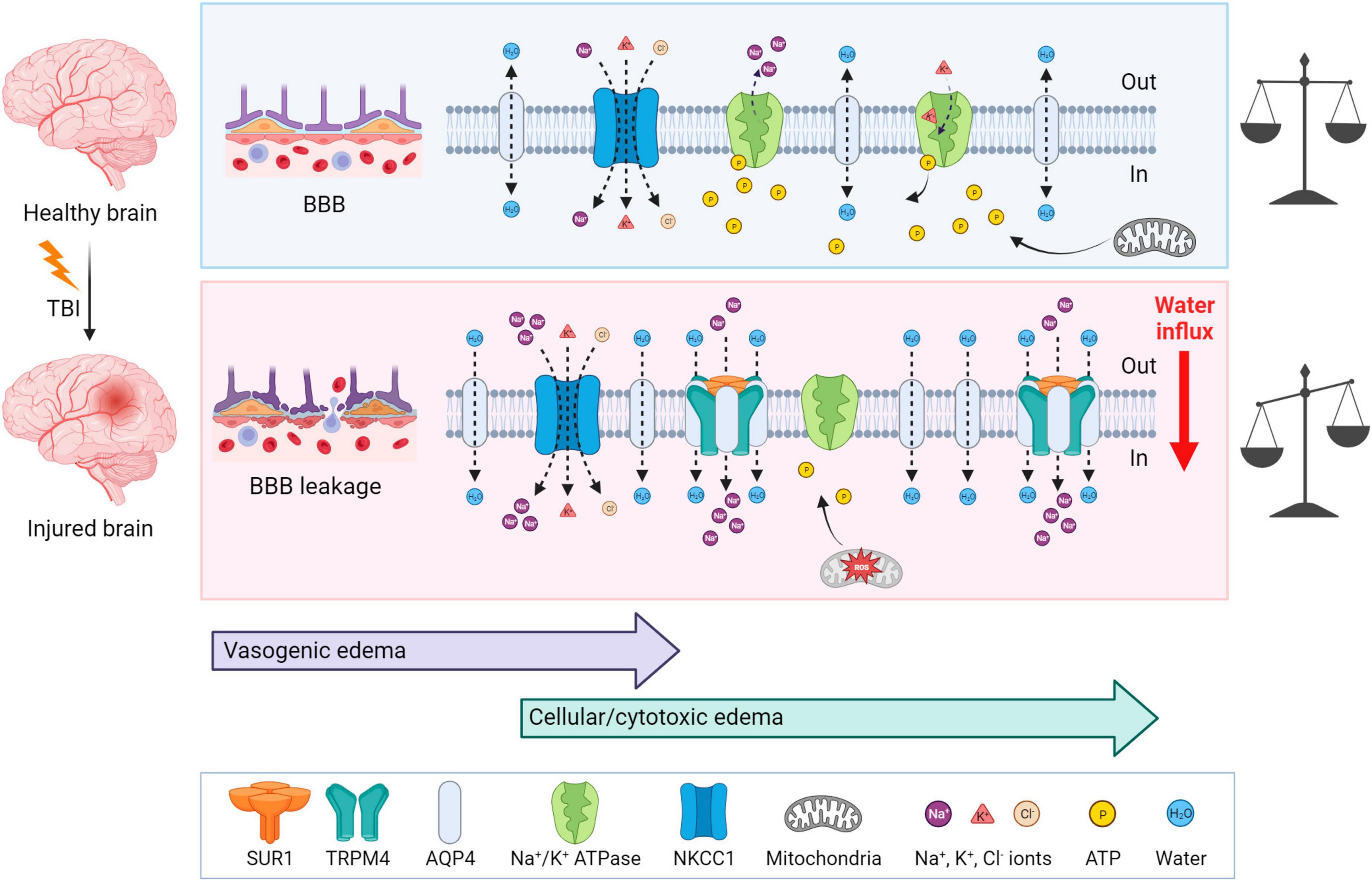
Figure 4. Mechanisms of brain edema development after traumatic insult—a comparison with a healthy brain. Upper panel: In physiological conditions, ionic balance is ensured by active or passive transport via channels, and mitochondria produce enough ATP to secure normal cellular function. Lower panel: At first, TBI induces BBB disruption and vasogenic edema, which is followed by cytotoxic edema with a slower onset. After TBI, AQP4, SUR1, and TRPM4 are upregulated and create a complex which contributes to astrocytic swelling by the intracellular transport of Na+ and H2O. NKCC1 during TBI is more activated and transports additional ions into the cells, particularly Na+. Moreover, due to the mitochondrial dysfunction induced by ROS, ATP is depleted. Lack of ATP inhibits the active transport via Na+/K+ ATPase and also terminates the ATP-dependent inhibition of SUR1-TRMP4 complex that transport Na+ ions. These events cause water influx and energetic and ionic disbalance, which can consequently result in cell death. TBI, traumatic brain injury; BBB, blood-brain barrier; NKCC1, Na+, K+, Cl– cotransporter; ROS, reactive oxygen species; SUR1, sulfonylurea receptor 1; TRPM4, transient receptor potential melastin 4. Created with BioRender.com.
Cytotoxic edema is characterized by cellular swelling, which arises from an intracellular influx of ions and water. The astrocytes express various channels contributing to water and ion homeostasis, such as aquaporins, NKCC1 (Na+, K+, Cl– cotransporter), Na+/K+ ATPase, or SUR1-TRPM4 (Sulfonylurea receptor 1–transient receptor potential melastin 4) channels (Figure 4). ATP-dependent ion pump functionality is tightly connected with ATP availability. During TBI, generated reactive oxygen species (ROS) cause mitochondrial dysfunction leading to impairment in ATP synthesis and thus to ATP depletion. Moreover, ATP is released from cells into the extracellular space immediately following TBI, therefore exacerbating the energetic disbalance (Moro et al., 2021). The authors also demonstrated that this ATP release can be slowed down significantly by blocking P2Y1 receptors or store-operated calcium channels (Moro et al., 2021). Hence, the ATP-dependent Na+/K+ pump, creating membrane potential by maintaining the Na+/K+ difference across the membrane, fails in providing transport of the ions, causing a change in the membrane potential, followed by an increase in osmolarity and water influx into the cells (Zusman et al., 2020).
Aquaporin-4 (AQP4), a channel participating in water homeostasis and transport, is also considered to be an important contributor to cytotoxic edema. AQP4 is predominantly expressed by astrocytes and enriched in their endfeet in contact with blood vessels (Neely et al., 2001; Amiry-Moghaddam et al., 2004). TBI causes the upregulation of AQP4 expression, which consequently induces the swelling of astrocytes (Kapoor et al., 2013). The degree of edema is dependent on the subcellular localization of AQP4; translocation of AQP4 mediated by calmodulin leads to increased water flux and astrocytic swelling (Kitchen et al., 2020). In AQP4 knockout mice, the migration of astrocytes to the injury site was reduced (Saadoun et al., 2005). Additionally, in wild-type astrocytes, AQP4 is polarized to the leading edge of the membrane and astrocytic migration can be enhanced by the extracellular osmotic gradient. Therefore, authors suggested, that AQP4 is involved in astrocytic cell migration toward the damaged areas after TBI (Saadoun et al., 2005).
Other contributors to astrocytic swelling include the increased activation of NF-κB (Nuclear factor κB) (Jayakumar et al., 2014) and the increased activation of NKCC1 cotransporter in astrocytes, which controls the transport of Na+, K+ and Cl– (Jayakumar et al., 2011). SUR1 is an ATP-binding part of various ion channels, serving as their regulatory subunit. SUR1 is associated with pore-forming subunits, such as Kir6.2 (ATP-sensitive potassium channel) or TRPM4 (ATP- and calcium-sensitive non-selective cation channel) (Chen et al., 2003). Interestingly, SUR1-TRPM4 is expressed in injured tissue, but not under physiological conditions (Chen and Simard, 2001; Chen et al., 2003). In human patients with post-traumatic brain contusions, overexpressed SUR1 was found in both astrocytes and microglia, and overexpressed Kir6.2 was observed in astrocytes (Martinez-Valverde et al., 2015; Castro et al., 2019). Additionally, upregulated SUR1, TRPM4, and Kir6.2 were discovered in both TBI patients and a CCI model of TBI (Gerzanich et al., 2019). ATP binding to SUR1-TRPM4 blocks its activity. Thus, ATP depletion leads to channel opening with consequential cation (predominantly Na+) influx, cell depolarization, and cellular swelling (Chen and Simard, 2001). Stokum et al. (2018) demonstrated an assembly of the SUR1-TRPM4-AQP4 complex in a murine model of brain edema using cerebellar cold injury (Stokum et al., 2018). Under physiological conditions, AQP4 creates a complex with other Na+ ion channels, such as TRPV4 (transient receptor potential cation channel subfamily V member 4) (Benfenati et al., 2011) or Na+/K+ ATPase (Illarionova et al., 2010). In the pMCAo (permanent middle cerebral artery occlusion) model of cerebral ischemia, loss of the AQP4-TRPV4 complex in double knockout mice leads to a reduction in the cytotoxic edema/ischemic lesion during the ischemic acute phase (Sucha et al., 2022). Overall, research in AQP4 and its complexes imply, that AQP4 and other channels, such as TRPV4 or TRPM4, may play a crucial role in cellular swelling and the extent of edema development in pathological states associated with AQP4/TRPV4/TRPM4 overexpression (Chmelova et al., 2019; Gerzanich et al., 2019; Sucha et al., 2022).
Following TBI, upregulated SUR1-TRPM4 and AQP4 assemble as a heteromultimeric water/ion channel complex, where SUR1-TRPM4 activity generates an osmotic pressure and causes water influx via AQP4 (Stokum et al., 2018; Figure 4). Interestingly, SUR1-Kir6.2 works contrarily to the SUR1-TRPM4 channel upon ATP depletion; activation of SUR1-Kir6.2 is followed by an outflux of K+ and hyperpolarization of the astrocytic membrane (Simard et al., 2012). To the best of our knowledge, there are no published studies explaining how TBI causes SUR1-Kir6.2 upregulation and which consequences it may cause, but some information is available from ischemia research. In the ischemic pMCAo model, SUR1-Kir6.2 was shown not to participate in cellular swelling while the SUR1-TRPM4 activity contributed to cytotoxic edema development (Woo et al., 2020). Additionally, some articles suggest the contribution of SUR1-Kir6.2 in a better outcome after hypoxia/ischemia (Yamada and Inagaki, 2005; Li et al., 2013).
Furthermore, the microglial immune response to TBI contributes to edema by the induction of AQP4 overexpression. Necrotic neurons from damaged tissue release HMGB1 (high-mobility group box protein 1) which in turn activates microglial TLR4 (Toll-like receptor 4). This event triggers a release of interleukin-6 (IL-6) from microglia, and as a result, it increases astrocytic AQP4 expression (Laird et al., 2014).
3.2 Excitotoxicity
Excitotoxicity is the process of exaggerated activation of the neuronal amino acid receptors (e.g., NMDA, AMPA, or KA receptors) by their excessive exposure to neurotransmitters, such as glutamate. Consequently, the influx of extracellular Ca2+ into neurons occurs, where Ca2+ may eventually trigger apoptotic signals through calpain mediating p53 induction and following caspase3-dependent neuronal apoptosis (Sedarous et al., 2003; Figure 5). Moreover, the Ca2+ influx induces neuroinflammation via NLRP3 (NLR family pyrin domain containing 3) and caspase-1. NLRP3 inflammasome is an intracellular sensor that detects microbial motifs and endogenous danger signals, providing a fast immune response (Kelley et al., 2019; Johnson et al., 2023). It is a complex composed of three protein subunit types: NLRP3 (a sensor protein), ASC (the adapter protein apoptosis-associated speck-like protein containing a CARD; caspase recruitment domain), and caspase-1. Besides altered calcium signaling, NLRP3 inflammasome is activated by other signals after TBI, including ionic changes (e.g., potassium and chloride efflux), or presence of extracellular ATP and ROS (O’Brien et al., 2020). After activation, NLRP3 inflammasome allows self-cleavage of pro-caspase-1 to active caspase-1, which consequently produces IL-1β or IL-18 (Johnson et al., 2023). The overexpression of IL-1β and IL-18 is followed by neuroinflammation that can lead to neuronal injury, apoptosis, or necrosis (Dong et al., 2009; Abdul-Muneer et al., 2017). Activation of NLRP3 inflammasome can also lead to cell death via pyroptosis (O’Brien et al., 2020; Figure 5).
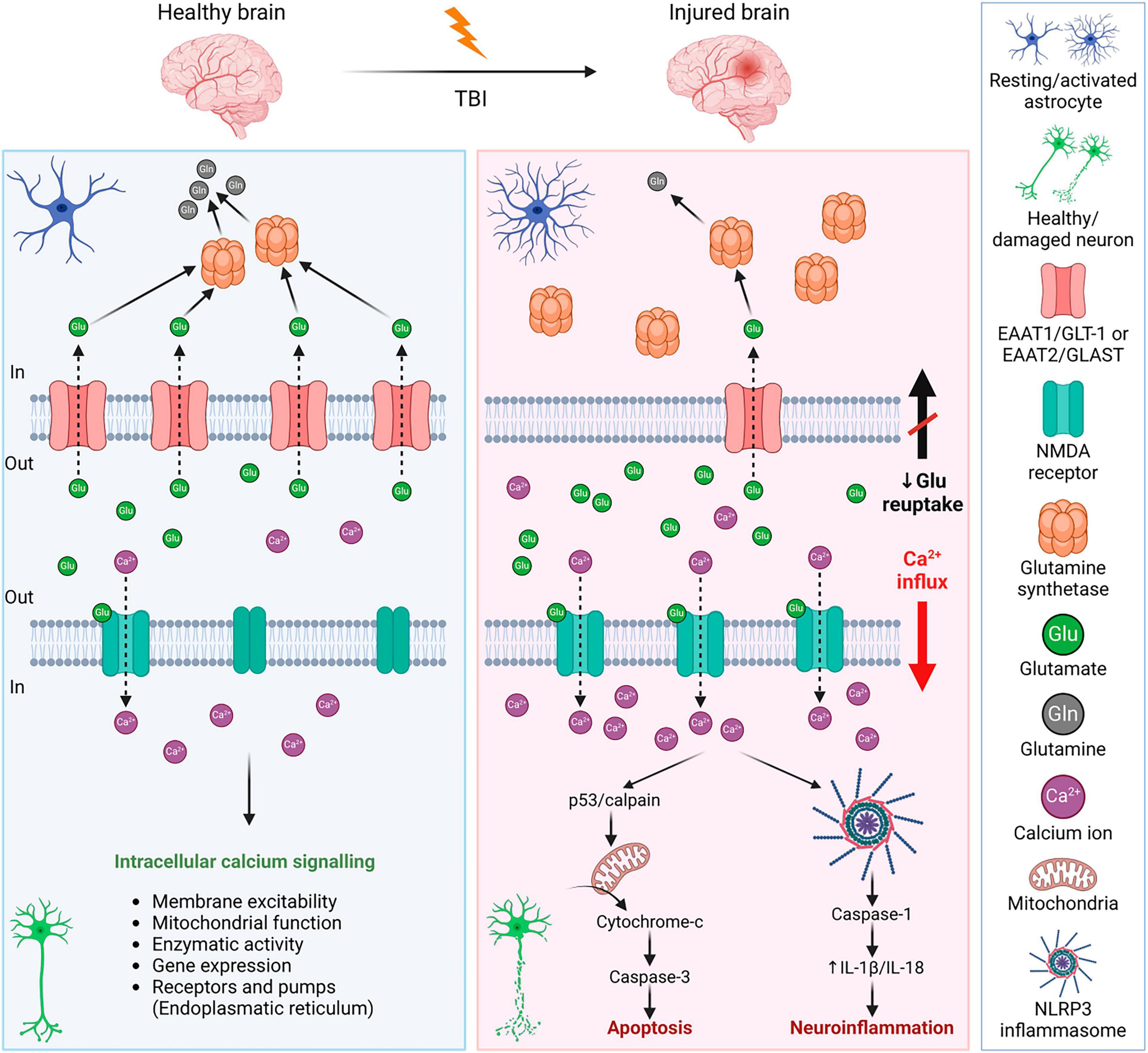
Figure 5. Mechanisms of glutamate excitotoxicity after traumatic brain injury—a comparison with a healthy brain. Left panel: In a healthy brain, the excess glutamate is cleared by astrocytes, where glutamate is converted into glutamine by glutamate synthetase. It is a strictly balanced environment to prevent neuronal death and neuroinflammation. Right panel: After TBI, the concentration of glutamate in the extracellular space increases due to disrupted BBB, or reduced glutamate reuptake due to the decreased expression of astrocytic glutamate transporters. Increased activation of neuronal NMDA receptors by glutamate binding evokes an influx of Ca2+ into the cells. Excessive Ca2+ triggers apoptotic signals leading to cell death and a release of IL-1β that induces immune response. For further details see text. NMDA receptor, N-methyl-D-aspartate receptor; IL-1β, interleukin 1β; BBB, blood-brain barrier; EAAT1/2, excitatory amino acid transporter 1/2; GLT-1, glutamate transporter 1; GLAST, glutamate aspartate transporter; NLRP3, NLR family pyrin domain containing 3. Created with BioRender.com.
After TBI, levels of extracellular glutamate increased in both patients and experimental models of TBI (Palmer et al., 1993; van Landeghem et al., 2001; Chamoun et al., 2010; Sowers et al., 2021). The excess glutamate contributes to secondary injury and increases the extent of the damage. There are different causes contributing to the elevated extracellular concentration of glutamate, which include increased release (disrupted BBB, dysregulated exocytosis) or decreased reuptake by glutamate transporters. Through glutamate transporters, both astrocytes and microglia participate in glutamate clearance. In the brain, there are several subtypes of glutamate transporters; glutamate transporters EAAT1/GLT-1 and EAAT2/GLAST are predominantly astrocytic, but they are present in microglia as well. Other glutamate transporters (EAAT3-5) are predominantly neuronal. In human patients with TBI, a decreased expression of EAAT1/GLT-1 and EAAT2/GLAST was observed (van Landeghem et al., 2006; Beschorner et al., 2007), which supports the idea of the involvement of glutamate transporters in the tissue damage and final outcome after TBI. Therefore, many studies have focused on glutamate metabolism and glial glutamate transporters.
Lehmann et al. (2009) used cultured cortical astrocytes to study the influence of high glutamate levels on the expression of glutamate transporters and glutamine synthetase (GS), which converts transported glutamate into glutamine. In their study, astrocytic expression of both EAAT1/GLT-1 and EAAT2/GLAST decreased in high glutamate medium (0.5–20 mM) via glutamate receptor-independent mechanism. On the contrary, an increase in GS expression was induced by higher levels of glutamate (≥1 mM) (Lehmann et al., 2009). A significant decrease in the protein expression of glutamate transporters was reported in the ipsilateral cortex during the acute phase following CCI in rats (Rao et al., 1998). In this model, ramified microglia with EAAT2/GLAST expression were present as early as 2 h post-TBI. From 4 up to 72 h after CCI, microglia expressing EAAT1/GLT-1 and EAAT2/GLAST were present in all observed areas, including the cortex, hippocampus, and lateral thalamus (van Landeghem et al., 2001). Yi et al. (2005) studied splice variants GLT-1v and GLT-1α in a rat model of lateral FPI. They observed a significant loss of GLT-1v (c-terminal splice variant) in the cerebral cortex (6–24 h post-TBI) and a transient decrease in the hippocampus and thalamus (6 h post-TBI). Interestingly, there was no significant change in GLT-1α in the cortex, but an increase was observed in the hippocampus (6–24 h post-TBI) (Yi et al., 2005). Additionally, it was found that GFAP (which is overexpressed after TBI) may modulate astrocytic glutamate transport in a region-dependent manner and be involved in EAAT2/GLAST trafficking (Hughes et al., 2004).
3.3 Neuroinflammation
Following TBI, the immune response is a natural process, which occurs within minutes post-injury. Although neuroinflammation is a necessary reaction to injury, its persistence causes long-term complications in TBI patients. Chronic neuroinflammation not only contributes to tissue damage but there is also growing evidence showing it as a main feature of other brain pathologies, such as dementia or Alzheimer’s disease (AD) (Eikelenboom et al., 2010; Perry et al., 2010). TBI itself is therefore considered a risk factor for AD and dementia development later in life (Plassman et al., 2000; Fleminger et al., 2003; Li et al., 2017), as persistent microgliosis is a common factor of these conditions.
Both microglia and astrocytes are involved in neuroinflammation, and their interplay is essential (Figure 6). Following TBI, microglia are activated by PAMPs (pathogen-associated molecular patterns) or DAMPs (damage-associated molecular patterns) released from damaged tissue (Jassam et al., 2017; Johnson et al., 2023). The process of microglial activation is complex and heterogeneous, depending on the severity, type of injury, and the extent of the damaged area and structure of the brain (Shields et al., 2020). In general, microglia express receptors recognizing cytokines, chemokines (e.g., CD86, CD206), and after activation, also major histocompatibility complex class II (MHCII). Microglia are activated by pro-inflammatory cytokines such as interferons (INF-γ), interleukins (e.g., IL-6), or tumor necrosis factor (TNF-α). Additionally, a fast microglial response is supported by ATP, released from damaged tissue, and by activated astrocytes (Davalos et al., 2005). Reactive astrocytes also contribute to microglial activation by producing cytokines, chemokines, nitric oxide (NO), or matrix metalloproteinase 9 (MMP-9). Furthermore, astrocytes, together with oligodendrocytes, secrete IL-33, which promotes the recruitment of microglia and macrophages post-TBI (Wicher et al., 2017). The production of inflammatory cytokines is mediated by TLR4 via TLR4/NF-κB pathway. During TBI, upregulation of TLR4 can be observed in hippocampal astrocytes and neurons and its depletion suppresses the production of pro-inflammatory cytokines IL-6, IL-1β, and TNF-α. Other pathways involved in signaling during neuroinflammation include JAK/STAT (Janus kinase/Signal transducers and activators of transcription), HMGB1, MAPK (Mitogen-activated protein kinase), or PPAR-γ (peroxisome proliferation-activated receptor γ). They are discussed in more details below.
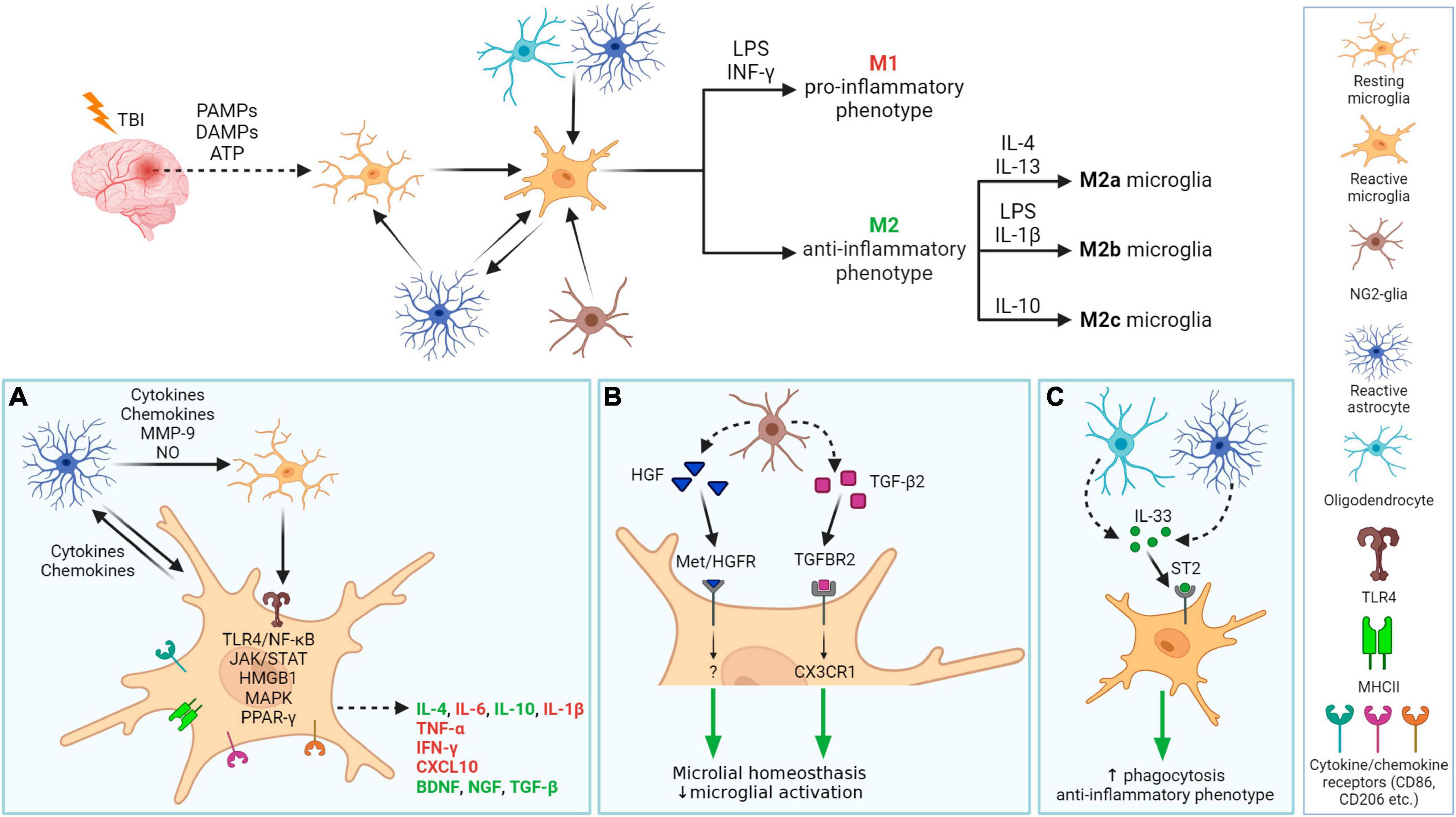
Figure 6. Neuroinflammation processes in damaged tissue after traumatic brain injury with a focus on glial cells. After TBI, PAMPs, DAMPs, and ATP are released from damaged tissue, which together with molecules (cytokines, chemokines) released by astrocytes, activate microglia. Activated microglia migrate into the damaged area and release pro- or anti-inflammatory factors. Microglial phenotype M1 and M2 (M2a-c resp.) phenotypes can be specifically activated. Other glial cells also have a role in neuroinflammation (A–C). (A) In damaged tissue, cellular communication between activated astrocytes and resting or activated microglia occur. Activated microglia receive signals via their receptors that trigger one of the cascades, e.g., ligand binding to TLR4 induces NF-κB dependent pathway that evokes the release of IL-6, IL-1β, or TNF-α.. Released molecules can have pro-inflammatory (red) or anti-inflammatory properties (green). (B): Microglial neuroinflammation activity can be regulated by NG2-glia, by releasing HGF of TGF-β2. (C) Both astrocyte and oligodendrocytes release IL-33, which promotes microglial anti-inflammatory phenotype and phagocytic ability. PAMPs, pathogen-associated molecular patterns; DAMPs, damage-associated molecular patterns; HGF, hepatocyte grow factor; TGF-β2, transforming grow factor β2; INF-γ, interferon γ; LPS, lipopolysaccharide; IL, Interleukin, e.g., IL-4; TLR4, toll-like receptor 4; MHCII, major histocompatibility complex class II; CD, cluster of differentiation, e.g., CD86; ST2, receptor suppression of tumorigenicity 2, also known as IL1RL1 or IL33R; interleukin-1 receptor-like 1, or interleukin-33 receptor; Met/HGFR, hepatocyte growth factor receptor encoded by the MET gene; TGFBR2, transforming growth factor beta receptor 2; CX3CR1, C-X3-C motif chemokine receptor 1; MMP-9, matrix metalloproteinase 9; NO, nitric oxide; TNF-α, tumor necrosis factor α; CXCL10, C-X-C motif chemokine ligand 10; BDNF, brain-derived neurotrophic factor; NGF, nerve growth factor; NF-κB, nuclear factor κB; JAK/STAT, janus kinase/signal transducers and activators of transcription; HMGB1, high-mobility group box 1; MAPK, mitogen-activated protein kinase; PPAR-γ, peroxisome proliferator-activated receptor γ. Created with BioRender.com.
Galectins act as master regulators in the inflammatory response associated with neurodegenerative disease development (Soares et al., 2021). Yip et al. (2017) found that activated microglia release galectin-3 as a TLR4 ligand, crucial for the full microglial response to proinflammatory stimuli, like lipopolysaccharide (LPS) (Yip et al., 2017). Furthermore, galectin-3 influences the inflammatory response to α-synuclein (α-syn) in microglial cells and act as a natural ligand of TREM2 (Triggering receptor expressed on myeloid cells 2) receptor involved in AD pathogenesis (Boza-Serrano et al., 2014; Qin et al., 2021). The role of galectin-3 has been intensively studied in ischemia and tumorogenesis, where it was shown to activate or modulate other signaling molecules and regulators of cellular processes such as interleukin kinase or tyrosine kinases (Wesley et al., 2013; Porebska et al., 2021). However, several studies indicate a significant early increase in galectin-3 expression also in various trauma models, including experimental TBI (Natale et al., 2003; Venkatesan et al., 2010). Yip et al. (2017) discovered significant galectin-3 expression in mouse cerebrospinal fluid (CSF) 24 h post-injury, likely stemming from a disrupted BBB (Yip et al., 2017). Galectin-3-dependent-TLR4 activation may contribute to prolonged microglia activation and sustained brain inflammation (Burguillos et al., 2015). However, another study revealed a positive correlation between human plasma galectin-3 levels and higher GCS scores (Shen et al., 2016). These findings suggest the potential role of galectin-3 as a biomarker in TBI, indicating a need for further exploration for clinical applications. In contrast, the other member of galectin family, galectin-1, suppresses activation markers, proinflammatory cytokines, and inducible nitric oxide synthase (iNOS) expression in microglia in inflamed CNS tissues, while its absence prompts classical microglial activation (Aalinkeel and Mahajan, 2016).
Following activation, microglia undergo polarization—they change their morphology into larger rounded ameboid cells, proliferate and migrate into the damaged region (Caplan et al., 2020). Activated microglia also start to release factors with pro-inflammatory/anti-inflammatory functions. The basic microglial phenotypes after activation can be distinguished into two types–the M1 type and the M2 type. M1 type is considered a pro-inflammatory phenotype, preferably producing pro-inflammatory compounds such as cytokines TNF-α, INF-γ, IL-1β, chemokines (e.g., C-X-C motif chemokine ligand 10, CXCL10), or ROS. Alternatively, the neuroprotective M2 phenotype produces anti-inflammatory molecules, such as interleukin IL-10 (which can also be expressed by the M1 phenotype, but to a lower extent) (Mills et al., 2000; Ziebell and Morganti-Kossmann, 2010). The M2 phenotype has 3 subtypes: M2a, M2b, and M2c, which differ in function, activation factor, and expression of specific markers (Zhang et al., 2022a). Microglial phenotype can be specifically activated: IFN-γ and LPS stimulate M1 phenotype; IL-4 and IL-13 trigger M2a phenotype; M2b phenotype is the result of LPS or IL-1β activation and IL-10 triggers M2c phenotype (Figure 6). Each of these phenotypes has a specific proteomic profile (Xu et al., 2017; Vergara et al., 2019). Notably, transcriptomic analysis of activated microglia revealed the limitations of these classifications—activated microglia rarely fit the classification perfectly; usually a mixed microglial population is observed. Furthermore, microglial gene expression is dynamic and time-dependent. Izzy et al. (2019) demonstrated the reduced ability of microglia to sense tissue damage in the early stage of TBI, which in a later stage (14 days post-injury, dpi) began to transfer into a specialized inflammatory state, with changes of IL-4, IL-10, and IFN-γ gene expression within 14–60 dpi (Izzy et al., 2019). Due to this diversity, Paolicelli et al. (2022) oppose dual nomenclatures, such as M1/M2 or pro- vs. anti-inflammatory microglia. Instead of restricting to these types, the authors advise using descriptions of phenotypes and transcriptome (Paolicelli et al., 2022).
Until recently, it was thought that only astrocytes and microglia contribute to neuroinflammation. However, recent studies have revealed the important role of NG2-glia in the brain immune system as well as its involvement in neuroinflammation (Figure 6). Nakano et al. (2017) reported that the elimination of NG2-glia activates the IL-1β pro-inflammatory pathway resulting in defects of hippocampal neurons due to neuroinflammation. Furthermore, NG2-glia-expressed hepatocyte growth factor (HGF) acts as a regulator of the neuroinflammation level (Nakano et al., 2017). According to Zhang et al. (2019), transforming growth factor beta receptor 2 (TGF-β2) expressed by NG2-glia regulates the microglial chemokine receptor CX3CR1 (C-X3-C motif chemokine receptor 1) via increased phosphorylation of the transcriptional modulator mothers against decapentaplegic homolog 2 (SMAD2) and therefore controls microglial homeostasis. In any case, the deficiency of NG2-glia in the Parkinson’s disease (PD) mouse model contributed to neuroinflammation (Zhang et al., 2019). These studies suggest that NG2-glia may have a role in neuroinflammation regulation in both physiological and pathological states.
3.4 Cell death, demyelination, and white matter degradation
Cellular death typically accompanies TBI and was observed in both TBI patients and experimental models. Cellular death was documented in several brain regions, including the cortex, hippocampus, and thalamus (Clark et al., 1997; Conti et al., 1998; Fox et al., 1998). Apoptosis following TBI is triggered by a caspase-dependent pathway (Yakovlev et al., 1997; Clark et al., 2000) or a caspase-independent pathway, where apoptosis is induced by several mitochondrial proteins, e.g., mitochondrial apoptosis-inducing factor AIF (Zhang et al., 2002). Apoptosis is region dependent, with an early response in white matter (12 h post-TBI) and a delayed apoptosis peak in the thalamus (2 weeks post-TBI) (Conti et al., 1998). Neurons and oligodendrocytes in white matter are more vulnerable to apoptosis following TBI than the rest of the cells in the brain. Loss of oligodendrocytes is supplied by NG2-glia proliferation, which can be observed even months after injury (Dent et al., 2015), and the differentiation into oligodendrocytes can be driven by myelin damage (Hill et al., 2014). Johnson et al. (2013a) found ongoing white matter degradation in the corpus callosum, and persistent neuroinflammation in patients 3 months post-injury (Johnson et al., 2013a). Flygt et al. (2016) observed oligodendrocyte cell death and an increase in the number of oligodendrocyte progenitor cells/NG2-glia in patients with moderate to severe TBI (Flygt et al., 2016). Oligodendrocyte cellular death is caused by several factors—the microglial release of inflammatory cytokines, such as TNF-α or IFN-γ, higher oxidative stress, increased levels of extracellular ATP and/or excess of glutamate, which commonly occurs in TBI tissue. Interestingly, Lotocki et al. (2011) demonstrated a positive effect of hypothermia on TBI-induced oligodendrocyte loss—a decrease in temperature after injury for 4 h led to suppression of caspase-3 activity, which consequently enhanced oligodendrocyte survival (Lotocki et al., 2011).
Since oligodendrocytes produce myelin, a decrease in the amount of myelin after TBI could be the result of oligodendrocyte apoptosis. Both white matter loss and demyelination can be detected in various brain regions. Damaged areas with atrophy include the corpus callosum (Johnson et al., 2013a; Green et al., 2014; Briggs et al., 2016), hippocampus (Green et al., 2014) pons or cerebellum (Spanos et al., 2007). TBI activates IKK/NF-κB (IKK, inhibitory-κB kinase activates NF-κB by phosphorylating IκBα, the inhibitory subunit of NF-κB) signaling in oligodendrocytes, which induces their senescence and is followed by disturbed myelination (Schlett et al., 2023). Mahoney et al. (2022) used magnetic resonance imaging to demonstrate that activated intracortical demyelination has a distinct spatial profile in comparison to the acute phase. Additionally, the impact of TBI-related demyelination manifests worse outcomes than age-related demyelination, where temporal, cingulated, and insular regions showed a higher degree of myelin loss (Mahoney et al., 2022). Demyelination is spontaneously followed by remyelination; this process has a protective effect ensuring axonal survival (Irvine and Blakemore, 2008). However, the remyelination process is limited by inflammation on the site of the demyelinated lesions and inadequate precursor differentiation.
Microglia, together with macrophages, remove dying cells, but the alleviation of inflammatory responses may also enhance apoptotic processes. According to Wang et al. (2020), microglial depletion promoted neurite growth and reduced the total neural apoptosis after the FPI model of injury (Wang et al., 2020). The microglial pro-inflammatory response can directly trigger apoptosis of oligodendrocytes; conversely the anti-inflammatory M2 phenotype of microglia support remyelination (Miron et al., 2013), therefore a balance between M1 and M2 phenotype seems to be essential for the remyelination process. Blocking of microglial NHE1 (Sodium-hydrogen antiporter 1), the protein responsible for NADPH oxidase and cytokine secretion in pro-inflammatory microglia, resulted in a reduced inflammatory response, and consequently promoted oligodendrogenesis and supported remyelination in TBI tissue (Song et al., 2022).
3.5 Reactive gliosis and scar formation
The formation of a scar following TBI is considered a defense mechanism, preventing the propagation of the tissue damage and the spread of toxic metabolites; however, it also prevents axonal growth leading to impaired neural function recovery (Silver and Miller, 2004; Burda and Sofroniew, 2014; Karve et al., 2016).
After TBI, it is plausible to divide the ensuing events into three phases, while the processes of these phases can overlap (Burda and Sofroniew, 2014; Figure 7). In the first phase (seconds to hours after injury, prolonged up to several days), trauma evokes acute cell death in the injury center. Immune cells are activated and recruited, including microglia. Microglia and NG2-glia migrate toward the damaged area, astrocytes do not migrate, but swell and can eventually become reactive. During the second phase (2–10 days after injury), cells, including scar-forming astrocytes, proliferate. During this phase, a lesion core containing fibroblasts, pericytes, or inflammatory cells, starts to form. The core is surrounded by a layer of reactive astrocytes separating the core and the peri-lesion perimeter, which contains reactive glial cells, and gradually transitions to healthy tissue. Tissue remodeling appears in the third phase (which can occur 7 dpi) and the lesion matures. The result is a persisting fibrotic and astrocytic scar. Tissue remodeling is also present in the peri-lesion perimeter and can persist for months post-TBI (Burda and Sofroniew, 2014).
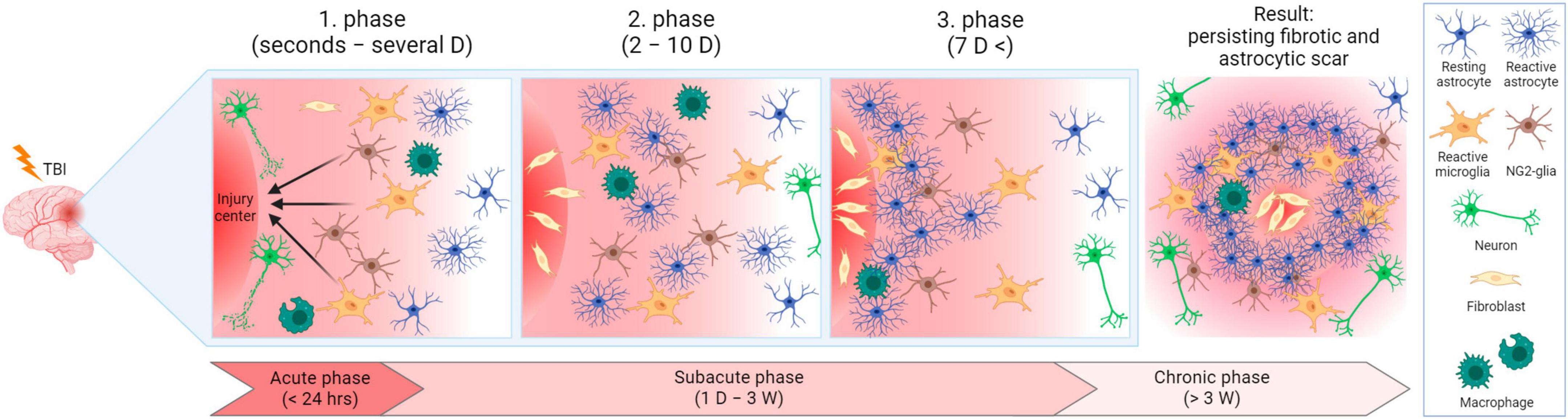
Figure 7. Lesion development after traumatic brain injury in time. In the acute phase, cells are damaged in the injury center, and immune cells are activated and recruited. Microglia and NG2-glia migrate toward the injured area and astrocytes become reactive. In the subacute phase, cells (including scar-forming astrocytes) proliferate. A layer of reactive astrocytes surrounds a forming lesion core. During the transition between the subacute and chronic phases, tissue remodeling and lesion maturation occur, resulting in permanent fibrotic and astrocytic scars. D, day/days; W, week/weeks. Created with BioRender.com.
However, a mild diffused injury may result in gliosis without scar core formation (Shandra et al., 2019). In various articles, the term “glial scar” or “astroglial scar” is used for the whole lesion. Therefore, it was suggested not to use these terms because astrocytes are not mesenchymal or stromal cells, which form scars in other damaged tissues (e.g., skin or heart). Hence, “scar formation” occurring in the brain after TBI does not correspond to the widely used terminology from other research areas (Sofroniew, 2020). In contrast, other studies use the term “glial scar” only to describe the glial border of the scar since glial cells are not the main compounds of the lesion (Adams and Gallo, 2018).
NG2-glia together with microglia are the first glial cells to react to the injury, though microglia extend their processes faster than NG2-glia (Hughes et al., 2013). During injury, NG2-glia re-enter the cell cycle, which in combination with the shortening of the G1 phase, results in their proliferation and an increase of the NG2-glia population (Simon et al., 2011). OPCs, which belong to the NG2-glia population, are recruited via TLR-2/CXCR3 pathway signaling to the lesion site, where they differentiate into oligodendrocytes (Sanchez-Gonzalez et al., 2022). However, part of the NG2-glia form the glial border together with astrocytes and microglia, without any differentiation (Levine, 1994). Since the reduction of the NG2-glia population delays wound closure, it was suggested that accumulation of NG2-glia on the lesion site also has a positive effect on tissue regeneration (von Streitberg et al., 2021).
By releasing signaling molecules, including cytokines (TNF-α, IL-1β, IL-6) or IGF-1 (Insulin-like growth factor-1, in spinal cord injury), microglia stimulate the transformation of astrocytes into reactive astrocytes, which are a key element of the glial scar. Increased microglial proliferation reduces the lesion size and enhances functional recovery after spinal cord injury. On the contrary, microglial depletion evokes the suppression of astroglial STAT3 phosphorylation, a critical regulator of astrogliosis, resulting in impaired glial scar formation (Bellver-Landete et al., 2019; Zhou et al., 2023).
In astrocytes, TBI enhances proliferation, alters gene and protein expression and triggers cytoskeletal remodeling, resulting in hypertrophic astrocyte soma and processes. There are two essential proteins for astrocytic remodeling, GFAP and vimentin (Vim), which are both overexpressed post-TBI. GFAP and Vim are responsible for the assembly and extension of the intermediate filament inside the astrocytic processes, and astrocytes seem to suppress neurogenesis in the damaged tissue by producing these two proteins (Wilhelmsson et al., 2004). According to Jiang et al. (2018) expression of GFAP can be mediated by TLR4—the TLR4 deficit leads to suppression of GFAP upregulation and consequently repressed astrocytic activation (Jiang et al., 2018). Another important astrocytic protein, debrin, which is also overexpressed in the injured brain, controls scar formation via the regulation of membrane trafficking of crucial membrane receptors, such as β1-integrin (Schiweck et al., 2021).
Glial fibrillary acidic protein is frequently used as a marker of reactive astrocytes for immunofluorescence analysis. However, Escartin et al. (2021) do not consider GFAP as a reliable marker for evaluating the level of reactive astrogliosis, because different studies have shown that the level of GFAP in astrocytes varies (Shandra et al., 2019; Early et al., 2020; Escartin et al., 2021). Some studies even identified “GFAP-negative” astrocytic populations (Walz and Lang, 1998; Kofler et al., 2002; Xu, 2018). However, the GFAP expression level of “GFAP-negative astrocytes” may simply be under the detection limit, which is then observable due to injury-induced GFAP upregulation or caused by the masking of GFAP during the paraformaldehyde fixation of samples, specifically in gray matter (Walz, 2000). Nevertheless, GFAP is still a useful marker of reactive astrogliosis, although it is recommended to confirm the findings by the use of another marker.
Based on the type of upregulated proteins produced by activated astrocytes, Liddelow et al. (2017) suggested distinguishing two basic types of reactive astrocytes: A1 and A2, similarly, to the proposed microglia M1 and M2 phenotypes (Liddelow et al., 2017). The A1 phenotype includes neurotoxic astrocytes with upregulated pro-inflammatory factors, such as INF-γ, TGF-β, or IL-1α, or the activation of the NF-κB pathway, which have detrimental effects on the injured CNS. On the other hand, the A2 phenotype astrocytes display neuroprotective and anti-inflammatory effects, with upregulation of trophic factors BDNF (Brain-derived neurotrophic factor), VEGF (Vascular endothelial growth factor), or bFGF (Basic fibroblast growth factor) (Liddelow and Barres, 2017; Liddelow et al., 2017). Nevertheless, compounds produced by activated astrocytes may play both detrimental and protective roles. For example, IL-6 is a proinflammatory cytokine, that is overexpressed during TBI and has a key role in mediating neuroinflammation (Yang et al., 2013; Edwards et al., 2020; Ooi et al., 2022). In contrast, astrocytic overexpression of IL-6 is beneficial for wound closure and causes a decrease in oxidative stress and apoptosis in the TBI cryo-lesion model (Penkowa et al., 2003). However, upregulated IL-6 may also increase BBB permeabilization (Rochfort and Cummins, 2015) or promote cerebral edema (Xu et al., 2014). In recent years, studies using two-photon imaging and RNA-seq profiling have revealed much broader heterogeneity of the astrocytic population that does not fit with a simple A1/A2 type division (Adams and Gallo, 2018; Early et al., 2020). Therefore, Escartin et al. (2021) recommend describing the astrocytic phenotype in combination with transcriptome analysis to avoid strict classification (Escartin et al., 2021). Despite the above-mentioned objections, some of the new articles still use the A1/A2 division.
Newly proliferated and elongated astrocytes were found to be a part of the glial scar border (Wanner et al., 2013). Reactive astrocytes increase the production of CSPGs, which also become part of the lesion. The degree of astrogliosis is dependent on the distance from the insult area (Wanner et al., 2013), with the highest level of astrogliosis observed in the injury site (Castejon, 1998). Due to the wide astrocytic heterogeneity, it is still not perfectly clear how exactly each subpopulation responds to the TBI.
Since the formation of the lesion has long-term consequences for patient health, the cells and molecules contributing to the formation of the lesion are potential targets for the treatment of TBI. It is generally believed that one of the factors preventing full recovery of the damaged tissue is the barrier created by astrocytes that prevents axonal regrowth. Alternatively, Anderson et al. (2016) showed that by preventing astroglial scar formation, no axonal growth was restored in the injured spinal cord. Interestingly, levels of CSPGs, known inhibitors of axonal growth (Asher et al., 2001, 2002), were not significantly altered, indicating that other cells are replacing the astrocytic production of ECM post-TBI (Anderson et al., 2016). However, astrocytes are not the only glial cell type that limits recovery—the accumulation of NG2 cells in the lesion together with the NG2 overexpression also inhibits axonal growth in vitro (Levine, 1994; Chen et al., 2002; Tan et al., 2005). On the contrary, Yang et al. (2006) showed, that NG2-glia promoted axonal growth in vitro and provided an adhesive substrate for axonal growth cones both in vitro and in the developing corpus callosum. The authors also demonstrated that upregulation of NG2 expression does not inhibit axonal growth in vitro (Yang et al., 2006). These findings emphasize the need for further research, to better comprehend the specific mechanisms through which glial cells impact post-injury recovery, and to resolve the contradictory observations in this field.
4 Important signaling pathways and factors regulating tissue response after traumatic brain injury
Neuroinflammation, and later neurodegenerative processes, are regulated by a number of signaling molecules and pathways whose detailed description would be beyond the scope of this review. Selected pathways and factors that are particularly involved in TBI-induced tissue response are discussed in more detail here (Figure 8):
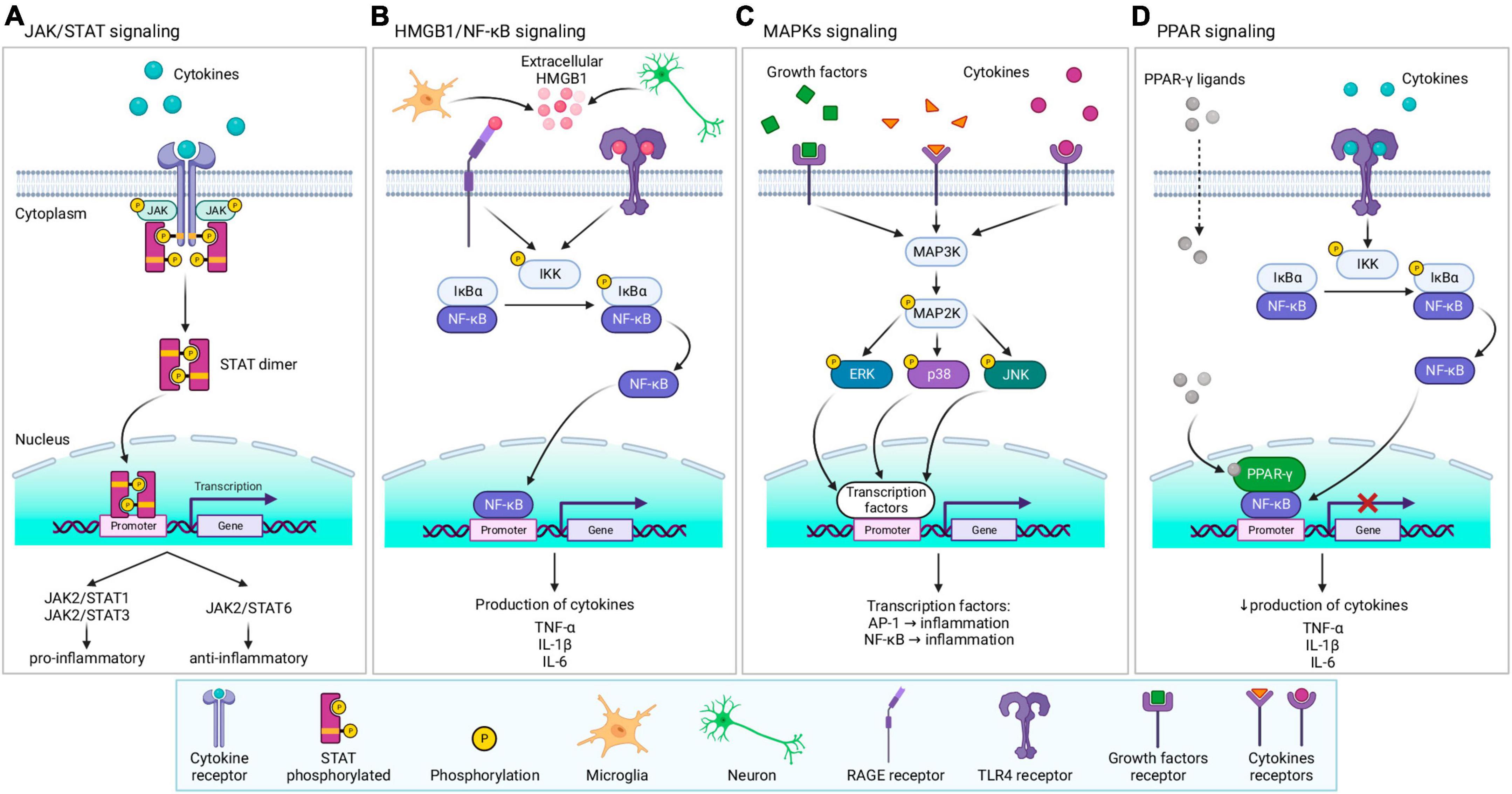
Figure 8. Overview of signaling pathways and factors involved in tissue response following TBI. (A) JAK/STAT signaling—after ligand binding to the receptor, JAK, and its downstream target STAT are activated. Activation triggers STAT dimerization, and translocation to the nucleus, where STAT dimer regulates the transcription of related genes, leading to the production of pro- or anti-inflammatory protein expression. (B) HMGB1/NF-κB signaling–extracellular HMGB1 released from microglia and neurons activates receptors (RAGE or TLR4), triggering the NF-κB pathway. IKK kinase phosphorylate IκBα, acts as an inhibitor of NF-κB which causes degradation of IκBα by the proteasome and translocation of NF-κB to the nucleus. After binding to the promoter, NF-κB regulates the transcription of related genes, leading to the production of cytokines. (C) MAPKs signaling—various ligands (cytokines, growth factors, etc.) can bind to specific receptors, triggering MAPKs cascade. Stimulation of MAP3K evokes activation of MAP2K, followed by activation of MAPKs (ERK, p38, or JNK) by phosphorylation. Activated MAPKs translocate into the nucleus and activate nuclear factors, including AP-1 or NF-κB, thereby regulating the transcription of related genes. (D) PPAR-γ signaling–specific ligands activate PPAR-γ, which consequently inhibits NF-κB, downregulating the transcription and decreasing inflammatory cytokines production. For further information see text. JAK, janus kinase;STAT, signal transducers and activators of transcription; HMGB1, high-mobility group box protein 1; NF-κB, nuclear factor κB; RAGE, receptor for advanced glycation end products; TLR4, toll-like receptor 4; IKK, inhibitory-κB kinase, also known as IκB kinase, IκBα, NF-κB inhibitor α; MAPK, mitogen-activated protein kinase; MAP3K, MAPK kinase; MAP2K, MAPK kinase; ERK, extracellular signal-regulated kinase; JNK, c-Jun N-terminal kinase; AP-1, activator protein 1; PPAR-γ, peroxisome proliferator-activated receptor γ; TNF-α, tumor necrosis factor α; IL, interleukin. Created with BioRender.com.
4.1 Janus kinase/signal transducers and activators of transcription (JAK/STAT)
Janus kinase is a family of cytoplasmic non-receptor tyrosine kinases, which are involved in signal transduction with various downstream targets (Figure 8A). Mammalian JAK comprises four members (JAK1-3 and tyrosine kinase 2, Tyk2). One of their many downstream targets are STATs, which are a family of intracellular transcription factors, including seven members (STAT1-4, STAT5a/b, and STAT6). Upon ligand binding (e.g., cytokines) to a specific receptor, JAK is recruited in close proximity to the receptor and is activated by phosphorylation. Consequently, downstream substrate STAT is also activated by phosphorylation, causing STAT dimerization and its translocation from the cytosol to the nucleus, where STAT regulates the expression of the related genes (Rane and Reddy, 2000; Xin et al., 2020; Jain et al., 2021; Li et al., 2022b). The JAK/STAT pathway is involved in cell proliferation, differentiation, apoptosis, and mediating innate and adaptive immunity (Rane and Reddy, 2000; Xin et al., 2020; Jain et al., 2021; Li et al., 2022a). Signaling via the JAK/STAT pathway promotes either the pro-inflammatory M1 phenotype (JAK2/STAT1 and JAK2/STAT3) or the anti-inflammatory M2 phenotype (JAK2/STAT6) (Li et al., 2022a). Cornel iridoid glycoside (CIG), which inhibits the JAK/STAT phosphorylation (JAK1/3, STAT1/3) in microglial murine cell line BV2, also inhibits microglial polarization toward M1 phenotype, promoting polarization to M2 phenotype (Qu et al., 2019).
In the pericontusional cortex of the rat WD model, levels of phosphorylated JAK2, STAT1, and STAT3 increased, with the highest detected level 3 h post-injury (Zhao et al., 2011). Wang C. et al. (2023) showed that Myricetin, a flavonoid able to attenuate levels of pro-inflammatory cytokines in vitro (Martinez-Coria et al., 2023), decreased levels of phosphorylated STAT1 and STAT3, and increased levels of EGFR (Epidermal growth factor receptor) and phosphorylated AKT (Protein kinase B) in Myricetin-treated microglia after LPS induction (Wang C. et al., 2023). The authors also found lower levels of IL-1β, IL-6, and TNF-α, and increased IL-4 and IL-10 levels in CCI animal models treated with Myricetin, in comparison to injured animals without treatment. Overall, Myricetin suppresses the pro-inflammatory response after TBI via the EGFR-AKT/STAT pathway (Martinez-Coria et al., 2023; Wang C. et al., 2023).
4.2 High mobility group box 1 (HMGB1)
High mobility group box 1 is a non-histone DNA-binding protein that has localization-dependent functions. Nuclear HMGB1 plays a role in gene expression, DNA repair, and chromosome stability, and cytoplasmatic HMGB1 induces autophagy. However, extracellular HMGB1 acts as a pro-inflammatory cytokine/trigger (Paudel et al., 2020; Dong et al., 2022). Following injury, including TBI, production of HMGB1 is upregulated, and can be released into the extracellular space by both neurons and glia (active release or due to cell death), which binds to receptors, such as RAGE (Receptor for advanced glycation end products) or TLR4 (Figure 8B). Receptor activation leads to the production of cytokines via the NF-κB pathway (Paudel et al., 2018, 2020; Manivannan et al., 2021; Dong et al., 2022).
In recent years, the emerging role of HMGB1 in TBI has revealed the potential of HMGB1 as both a biomarker and target for TBI treatment (Paudel et al., 2018; Manivannan et al., 2021; Li et al., 2022b). In a CCI mouse model, Webster et al. (2019) showed that levels of extracellular HMGB1 are age-dependent, with higher extracellular as well as serum levels of HMGB1 in juvenile animals (aged 3 weeks) than in adults (aged 8-9 weeks) (Webster et al., 2019). To our knowledge, there is no article exploring HMGB1 expression and localization in the aged population after TBI.
Additionally, HMGB1 upregulation induced by the NLRP3 inflammasome contributes to cognitive dysfunction in the chronic phase of TBI (Tan et al., 2021). Inhibition of HMGB1 translocation caused a reduction of edema and production of TNF-α and iNOS in an FPI model (Okuma et al., 2012). Targeting HMGB1 also led to lower expression of IL-1β, TNF-α, and IL-6, and contributed to edema reduction in a CCI model of TBI (Yang et al., 2018). Inhibition of HMGB1 or RAGE causes a decrease in the number of pro-inflammatory microglia and a shift toward anti-inflammatory phenotype in spinal cord injury (Fan et al., 2020).
4.3 Mitogen-activated protein kinases (MAPKs)
Mitogen-activated protein kinases are a family of serine/threonine protein kinases, that convert extracellular stimuli into various cellular responses. Members of MAPKs involved in neuroinflammation and expression of inflammatory cytokines, include MAPK subfamilies ERK (extracellular signal-regulated kinase); ERK (1-7), p38 (p38α, p38β, p38γ and p38δ), or JNK (c-Jun N-terminal kinase; JNK1-3) (Cuenda and Rousseau, 2007; Figure 8C). MAPKs are activated by phosphorylation upon diverse signals, including the presence of inflammatory cytokines and glutamate toxicity (p38 and JNK) or the presence of growth factors, oxidative stress, and an intracellular influx of calcium (ERK) (Roux and Blenis, 2004; Cargnello and Roux, 2011; Otani et al., 2011).
Following TBI, albumin can be released and activate astrocytes and microglia via MAPK signaling. In study of Ralay Ranaivo and Wainwright (2010), albumin-activated astrocytes showed elevated levels of phosphorylated ERK, p38, and JNK, which decreased after 24 h to levels comparable with controls. Albumin also evoked upregulated production of IL-1β, nitrite, iNOS, and CX3CL1, and downregulated production of S100B (S100 calcium binding protein B) in astrocytes. Inhibition of p38 MAPK or ERK also decreases astrocytic production of IL-1β, nitrite, and CX3CL1, but does not change the levels of S100B. Microglia exposed to albumin contained increased levels of phosphorylated ERK, p38, and JNK as well as more upregulated production of IL-1β and nitrite than controls. Inhibition of microglial p38 MAPK (but not ERK or JNK) enhanced the IL-1β levels even more (Ralay Ranaivo and Wainwright, 2010). According to Mori et al. (2002), trauma activated ERK and p38 MAPK, but not JNK in both in vitro and the CCI model. The authors also showed that only the inhibition of ERK signaling increased cell survival in vitro and led to a decrease in lesion volume in vivo; inhibition of p38 or JNK did not show any effects (Mori et al., 2002). However, in the lateral FPI model, increases in the levels of phosphorylated ERK and JNK were detected, but not p38 (Otani et al., 2002). These discrepancies may be caused by differences in methodology or detection, or due to differences between TBI models.
The main p38 MAPK isoform responsible for regulation of cytokine production, p38α, was shown to upregulate the expression of IL-1β and TNF-α in LPS-induced BV2 microglial cell line (Cuenda and Rousseau, 2007; Bachstetter et al., 2011). Similarly, deletion of microglial p38α led to diminished neuroinflammation, alterations in microglial morphology, and downregulation of pro-inflammatory factor expression (IL-1β, IL-6, TNF-α, CCL2, CXCL10) in p38α knockout mice after CCI (Morganti et al., 2019). Interestingly, knockout of p38α evoked a significant increase in cytokine levels 6 h post-injury, in comparison to the wild-type midline FPI model. However, at 7 dpi cytokine levels were elevated only in the injured wild-type (Bachstetter et al., 2013). This study suggested, that p38α may be responsible for balancing the inflammatory response—reducing microglial overreaction in the acute phase and sustaining microglial activation in the later TBI phases.
Since MAPKs have an important role in neuroinflammation, its members are already known targets for anti-inflammatory drugs. Li et al. (2022a) studied the effects of curcumin on suppressing inflammatory processes in a WD model. Curcumin administration caused a decrease of phosphorylated p38 and NF-κB, and consequently a decrease in expression of pro-inflammatory cytokines IL-1β, IL-6, and TNF-α compared to untreated animals after injury (Li et al., 2022a). Kodali et al. (2023), administered human mesenchymal stem cell-derived extracellular vesicles (hMSC-EV) intranasally to animals after CCI. The hMSC-EVs were incorporated into neurons and microglia; the treatment inhibited the activation of NLRP3 inflammasome in the acute phase and reduced the density of pro-inflammatory microglia in the chronic phase. The authors also focused on molecules involved in p38/MAPK signaling—the treated animals showed a significant decrease in levels of IL-6, MyD88 (Myeloid differentiation primary response 88), AP-1 (Activator protein-1), and IL-8 compared to the injured animals, but levels of p38 MAPK did not differ between treated and injured animals (Kodali et al., 2023). Another drug used for the regulation of postmenopausal osteoporosis, Bazedoxifene, showed neuroprotective properties via inhibition of the MAPK/NF-κB pathway in the CCI model. Bazedoxifene suppresses phosphorylation of ERK, p38, and JNK, attenuates brain edema, and decreases levels of IL-6, IL-1β, COX-2 (Cyclooxygenase 2), and TNF-α (Lan et al., 2019).
4.4 Peroxisome proliferation-activated receptors (PPARs)
Peroxisome proliferation-activated receptors are a family of nuclear receptors, which have three members (PPAR-α, PPAR-β/δ, and PPAR-γ). In inflammatory conditions, PPARs role is to suppress the activity of NF-κB, which regulates the activity of immune cells, reduces the production of anti-inflammatory compounds (e.g., TNF-α or IL-1β), or diminishes oxidative stress (Cai et al., 2018; Figure 8D). In the context of TBI, PPAR-γ is the most extensively studied; due to its important and relatively well-known role, PPAR-γ is a focal point in neuroinflammation therapy. With the exception of inflammation, PPAR-γ also plays a role in the regulation of adipogenesis, glucose homeostasis, cellular differentiation, and apoptosis (Stahel et al., 2008; Qi et al., 2010; Cai et al., 2018; Korbecki et al., 2019).
Deng et al. (2020) found decreased levels of PPAR-γ, and increased levels of pro-inflammatory/apoptotic factors (IL-6, caspase-3, NO) in human TBI patients, and a similar decrease in PPAR-γ levels in an animal CCI model (Deng et al., 2020). The same study showed that application of Pioglitazone (a drug used to treat diabetes) in injured animals increased levels of PPAR-γ in comparison to both injured non-treated and control animals. Pioglitazone treatment also decreased levels of IL-6, and phosphorylated NF-κB, reduced cerebral edema, and improved functional outcomes (Deng et al., 2020). Additionally, application with a PPAR-γ antagonist worsened the neuroprotective properties of Candesartan (Angiotensin II AT1-receptor blocker) in the CCI model; the PPAR-γ antagonist caused an increase in the number of activated microglia and partially diminished the positive effect of Candesartan in lesion volume reduction (Villapol et al., 2012). These data suggest that Candesartan also activates PPAR-γ. Wu et al. (2023) tested the effect of ω-3 polyunsaturated fatty acid administration on the WD model of TBI. The authors found reduced levels of NF-κB, IL-1β, IL-6, and TNF-α in the treated animals, as well as reduced edema. Additionally, both mRNA and protein levels of PPAR-γ increased and NF-κB decreased in treated animals post-TBI, in comparison to injured animals without treatment. These results suggest that the neuroprotective outcome of ω-3 polyunsaturated fatty acid therapy is partially caused by affecting the PPAR-γ/NF-κB pathway (Wu et al., 2023).
4.5 Glia maturation factor (GMF)
Glia maturation factor is a protein expressed in various organs and cell types. GMF has two isoforms: a CNS-specific isoform GMF-β (GMFB), expressed by neuronal and glial cells, and GMF-γ (GMFG), expressed predominantly by endothelial and inflammatory cells. Despite its name, GMFG does not act as a glial maturation factor (Walker, 2003; Ikeda et al., 2006; Fan et al., 2018).
GMFB is a pro-inflammatory protein that contributes to neuroinflammation following injury, including TBI. GMFB promotes reactive gliosis after TBI, and regulates the polarization of activated microglia, with favor toward pro-inflammatory phenotype (Yin et al., 2018; Ahmed et al., 2020).
In the model of cryogenic TBI, GMFB protein levels increased by 1 dpi, with a maximum level 14 dpi in the lesion area. The injury also elevated mRNA levels of GMFB 7 dpi, with a maximum at 14 dpi (Hotta et al., 2005). Ahmed et al. (2020) found that the absence of GMFB results in a reduction of lesion volume, an increase in neuronal survival, and inhibited gliosis after WD, compared to wild-type injured animals. In contrast with the wild-type WD model, activated microglia polarized to anti-inflammatory phenotype in GMFB knockout animals post-injury, with a decreased production of TNF-α and IL-6 and an increased expression of IL-4 and IL-10 (Ahmed et al., 2020). Similarly, GMFB deletion reduced neuroinflammation, decreased phosphorylation of NF-κB, and downregulated the production of GFAP, iNOS, and COX-2 compared to injured wild-type animals (Selvakumar et al., 2020).
Since GMFB is associated with neuroinflammation, researchers also explored the potential role of GMFB as a modulator of inflammatory signaling pathways. In vitro studies showed that overexpression of GMFB can activate p38, and NF-κB, slightly induce ERK, and have no effect on JNK activation (Zaheer and Lim, 1998; Zaheer et al., 2001, 2007). GMFB phosphorylated by PKA (proteinkinase A) activates p38 but inhibits the activation of ERK in vitro (Lim and Zaheer, 1996; Zaheer and Lim, 1996). However, the exploration of connections between GMFB and inflammatory signaling pathways requires more in-depth investigation, incorporating in vivo experiments into the research.
5 Reactive species and free radicals in TBI-induced tissue damage and neurodegeneration
Oxidative metabolism represents a notable metabolic advancement for aerobic organisms as it produces 36 ATP molecules from each glucose molecule in the presence of oxygen (Reiter et al., 2017). The mitochondrial electron transport chain (ETC) generates ATP but also leads to electron leakage, resulting in the production of superoxide anion radicals (•O2–). Under physiological conditions, 1–3% of oxygen is converted to •O2–. However, disruptions in ETC components during CNS pathologies increase electron leakage and elevate ROS generation (Hiebert et al., 2015; Reiter et al., 2017).
Reactive oxygen species comprise mostly of free oxygen radicals containing at least one atom of oxygen together with one or more unpaired electrons. Besides •O2– itself, ROS also include secondary reactive species generated by the reaction of •O2– with other molecules (Cornelius et al., 2013; Fridovich, 2013), for example hydrogen peroxide, hydroxyl, peroxyl and hydroperoxyl radicals. ROS are vital for some processes involving energy transfer, immune protection and signaling (Hakiminia et al., 2022). During physiological conditions, antioxidant defenses, involving enzymes superoxide dismutase (SOD), glutathione peroxidase (GPx), catalase (CAT), glutathione reductase (GR), and non-enzymatic elements (glutathione, vitamin E, vitamin C), serve as effective safeguards against oxidative threats (Fernandez-Gajardo et al., 2014; Mendes Arent et al., 2014). Oxidative stress occurs when oxygen and free radicals surpass the antioxidant system’s capacity (Lewen et al., 2000).
Following injury-induced excitotoxicity, excessive Ca2+ levels promote the generation of ROS and NO (Higgins et al., 2010). Elevated free radical concentrations result in the modification of key macromolecules such as DNA, proteins, and lipids, impairing various cellular processes (Valko et al., 2007). The reversible and irreversible changes in these macromolecules contribute to a spectrum of disorders, including neurodegenerative disease (Droge, 2002; Poprac et al., 2017). Following TBI induction, the injured nervous system experiences dynamic changes with the emergence of potential •O2– sources in the initial minutes and hours. These sources include enzymatic processes in the arachidonic acid (AA) cascade, autoxidation of biogenic amine neurotransmitters, “mitochondrial leak,” xanthine oxidase activity, and extravasated hemoglobin oxidation. Furthermore, NADPH oxidases (Nox), a family of membrane enzymes, actively convert oxygen into ROS. Nox enzymes significantly contribute to the pathophysiology of the nervous system, playing a pivotal role in the development of secondary injury following TBI (Angeloni et al., 2015). In the initial phase of TBI, an excess of ROS is predominantly generated by granulocytes and macrophages, rather than by activated or resting microglia, resulting in pronounced oxidative stress at the lesion site (Abe et al., 2018; Wu et al., 2022). During the chronic stage of TBI, microglia or macrophages assume a more prominent role in ROS production and oxidative stress within the injured brain, as evidenced by the augmented presence of Nox2 in microglia or macrophages 1 year post-injury (Loane et al., 2014).
Another significant category of reactive molecules constitute reactive nitrogen species (RNS), where Arginine (Arg) serves as their primary source. Arg is a semi-essential amino acid playing a crucial role as a precursor for metabolites such as ornithine, agmatine, creatine, and polyamines (Madan et al., 2018). Moreover, Arg metabolism, facilitated by nitric oxide synthases (NOS), generates NO and L-Citrulline. These metabolites contribute to diverse physiological functions, such as modulating cerebral blood flow, energy production, and tissue regeneration (Mader and Czorlich, 2022).
Depleting the L-Arg substrate can induce oxidative stress through the disconnection of NOS, resulting in the formation of oxygen radicals and potentially giving rise to the highly toxic oxidant peroxynitrite (ONOO–) (Cherian et al., 2004). NO possesses anti-inflammatory properties through redox regulation of the nuclear factor NF-κB (Reynaert et al., 2004). Consequently, the depletion of NO may intensify neuroinflammatory reactions. A decrease in Arg levels has been previously documented in the rat cortex 6 h after TBI using a WD model (Amorini et al., 2017). Furthermore, several clinical and preclinical studies have reported comparable changes in Arg metabolism lasting up to 3 dpi (Jeter et al., 2012; Zheng et al., 2020).
In experimental models, administering L-Arg after injury has demonstrated therapeutic benefits, including increased cerebral blood flow and reduced contusion volume. This positive effect is attributed to elevated NO production (Madan et al., 2018). However, literature on TBI presents conflicting roles of neuronal and glial NO. Inhaled NO increased cerebral blood flow and prevented ischemia causing secondary brain damage after TBI in mice, thus improving outcomes with prolonged exposure (Terpolilli et al., 2013). However, higher NO metabolites near neurons correlated with poorer survival in TBI patients (Tisdall et al., 2013). A placebo-controlled human trial with the NOS inhibitor VAS203 yielded better Glasgow Outcome Scale (categorizes the outcomes of patients after TBI) results at 6 months (Terpolilli et al., 2009). The inhibitor N(G)-nitro-L-arginine methyl ester (L-NAME), targeting all NOS forms, yielded mixed outcomes in TBI (Lu et al., 1997; Wada et al., 1999). These contradictions may result from spatial and temporal variations in NO production (Cherian et al., 2004). NO, generated from Arg by NOS isoforms, exhibits cell-specific expression: eNOS (endothelial) in vascular endothelium, nNOS (neuronal) in neurons, and iNOS (inducible) in macrophages and glial cells (Madan et al., 2018). Pharmacological investigations into NO’s role in TBI pathology have often used systemic approaches with L-Arg, a substrate for all NOS forms, or non-selective NOS inhibitors. However, such methods lack insights into the specific impact of L-Arg on distinct cell types or NO production from major cell-specific NOS isoforms (Madan et al., 2018).
Transcription factor Nrf2 (Nuclear factor erythroid-derived 2 related factor 2), acts as a regulator of both cytosolic and mitochondrial ROS production through NADPH oxidase, and is an important player in the oxidative stress-mediating tissue response following TBI (Kovac et al., 2015). Nrf2 signaling decreases with age in both humans and experimental animals, which suggests the possibility of an impaired response to oxidative stress in aged brains (Ngo and Duennwald, 2022).
Following activation by TBI-induced oxidative stress, Nrf2 is translocated from the cytoplasm into the nucleus, where it forms a dimer with transcription factor sMAF (small MAF protein; musculoaponeurotic fibrosarcoma) and then binds to ARE (Antioxidant response element, a cis-acting enhancer element), activating transcription of downstream targets, including heme oxygenase-1 (HO-1), glutathione S-transferase (GST), or SOD, which serve as protective enzymes against oxidative stress (Wu et al., 2022). Specifically, HO-1 catalyzes heme degradation that generates iron ions, biliverdin, and carbon monoxide (CO). In addition to the antioxidative role of biliverdin and CO, these products can also act as anti-inflammatory, anti-apoptotic, or anti-proliferative (Loboda et al., 2016). Morita et al. (2020) found an increased number of HO-1 expressing astrocytes (a significant increase in the number of HO-1-positive (HO-1+) cells at 1 dpi, with the maximum at 7 dpi) and microglia (delayed onset compared to astrocytes, maximum of HO-1+ cells at 14 dpi) in the CCI model (Morita et al., 2020). Furthermore, the NF-κB activity influences the ROS levels, by inducing the expression of both antioxidant (e.g., CAT, or HO-1) and pro-oxidant proteins (e.g., iNOS, or COX-2). ROS itself can influence NF-κB signaling at multiple levels of the pathway (both as an activator and inhibitor of the pathway) (Morgan and Liu, 2011).
Additionally, the cross-talk between Nrf2 and NF-κB is important for maintaining cellular homeostasis, because Nrf2 inhibits the NF-κB pathway and vice versa. Nrf2 and NF-κB compete for the co-activators of transcription, including the CREB binding protein (binding is dependent on the relative amount of Nrf2 and NF-κB in the nucleus), which can impact the balance and change the cellular inflammatory and stress response. The NF-κB p65 subunit can also directly inhibit the Nrf2 pathway. Interestingly, Rac-1 (Rac family small GTPase-1) activated NF-κB can induce Nrf2 and upregulate the expression of HO-1. Products of HO-1 then act as negative regulators of NF-κB inflammatory activity, by preventing NF-κB activation (Bellezza et al., 2018; Gao et al., 2021).
6 The role of exosomes and microRNA in reactive gliosis
The initiation and development of reactive gliosis is a complex process requiring precise communication between different cell types in which information is carried by the content of released extracellular vesicles (EVs). The family of EVs encompasses a diverse array of particles, including exosomes (30–100 nm diameter), microparticles (MPs; 100–1000 nm) and apoptotic bodies (2–5 μm) (Kumar et al., 2017). EVs have a dual role in cellular processes—they can be received by cells and simultaneously, they can be released from cells to transmit biological messages to neighboring cells. Exosomes are secreted by various cell types in the central as well as peripheral nervous system, including astrocytes (ADEs–astrocytes-derived exosomes), microglia (MDEs), oligodendrocytes (ODEs) and neurons (NDEs) (Potolicchio et al., 2005; Kramer-Albers et al., 2007; Thompson et al., 2016). The exosomes encapsulate diverse bioactive molecules such as microRNA (miRNA), mRNA and proteins (cytokines) and take part in intercellular crosstalk in both, physiological and pathological conditions (Kalluri and LeBleu, 2020).
In 2009, several expression profiling studies highlighted the potential role of miRNA in TBI (Lei et al., 2009; Redell et al., 2009). Notably, 136 miRNAs exhibited altered expression post-TBI, with changes evident across all four examined time points, except for miRNA-21. Bioinformatic analysis of validated miRNAs (miR-107, -130a, -223, -292-5p, -433-3p, -451, -541, and -711) regulated by TBI revealed an enrichment of proteins involved in diverse biological processes and functions typically initiated post-injury. These encompassed signal transduction, transcriptional regulation, proliferation, and differentiation (Redell et al., 2009). The list of miRNA with a known effect in TBI-induced gliosis can be found in Supplementary Table 2.
Interestingly, miRNA-21 expression and processing are governed by bone morphogenetic protein (BMP) and JAK/STAT signaling pathways, both of which have demonstrated a crucial role in regulating astrocytic responses (Setoguchi et al., 2004; Sahni et al., 2010; Kohanbash and Okada, 2012).
Sahni et al. (2010) revealed that the overexpression of miRNA-21 in cultured astrocytes induces a decrease in cell size, process thickness, and GFAP expression. These observations suggest a plausible role for miRNA-21 in modulating astrogliosis (Sahni et al., 2010). The upregulation of miRNA-21 expression is observed in astrocytes proximate to the lesion site following injury. Astrocytic hypertrophy decreases with miRNA-21 overexpression but increases with miRNA-21 inhibition, leading to more axon sprouting in the lesion during chronic injury stages (Bhalala et al., 2012). Targeting miRNAs may affect astrocytic hypertrophy, modulate glial scar progression and potentially improve functional recovery after brain injury.
7 The remote tissue response to traumatic brain injury
Glial responses to brain injury can be observed in a variety of brain structures and regions (Zhao et al., 2019). The following studies described TBI-evoked gliosis in the entorhinal cortex (Bolton and Saatman, 2014), hippocampus (Allen et al., 2000; Aungst et al., 2014; Bolton and Saatman, 2014; Luo et al., 2014; Zhao et al., 2017), corpus callosum (Bennett et al., 2012; Luo et al., 2014; Rodriguez-Grande et al., 2018; Zhao et al., 2019) and contralateral cortex (Miyazawa et al., 1995; Zhao et al., 2019).
In young mice, a single moderate TBI can result in long-lasting changes in astrocytes (GFAP and AQP4 staining) and microglia (Iba1 staining; Ionized calcium-binding adaptor molecule 1) in remote areas. Even 12 months after the damage, astrogliosis was still evident in the hippocampus; and both basal nuclei and the hippocampus continued to exhibit active microglia. Additionally, changes in the expression level and cellular location of the astrocytic protein AQP4 are dependent on the area. According to Obenaus et al. (2023), these findings have also been linked to cognitive deficiencies (Obenaus et al., 2023). The loss of AQP4 polarity brought on by TBI was demonstrated by Zhao et al. (2017) using the CCI mouse model. Even 4 weeks after the TBI, they observed astrogliosis in the contralateral hippocampal CA1 region. The GFAP-positive (GFAP+) reactive astrocytes soma and coarse processes are where AQP4 relocated, and this movement is controlled by adenosine A2A receptor signaling. As a result of decreased phosphorylated tau clearance, AQP4 seems to shift in polarity, which is followed with hyperphosphorylated tau protein accumulation in the CA1 hippocampus (Zhao et al., 2017).
The result of an injury may vary if the damage occurs in gray or white matter only or in both. Mattugini et al. (2018) identified and defined macrophage migration inhibitory factor (MIF) as a factor that regulates NG2-glia proliferation. The upregulation of MIF in injured gray matter supports the proliferation of NG2-glia, but not astrocytes and/or microglia. On the other hand, down-regulation of MIF is induced, if both gray and white matter are injured. The authors suggest that the proliferation of glial cells in gray matter is influenced by white matter injury. Of note, microglial reactivity was higher in white matter in comparison to gray matter (Mattugini et al., 2018).
The cerebellum is another remote region of the brain that can be affected by TBI. Some cortical brain injury models (FPI and CCI) result in the loss of neurons and activation of microglia and/or astrocytes in the cerebellum (Mautes et al., 1996; Park et al., 2006; Igarashi et al., 2007). Following recurrent cerebral TBI, glial response was also detected bilaterally in the brainstem and cerebellum (Bolton and Saatman, 2014).
8 Long-term impact of traumatic brain injury
In view of long-term consequences, TBI is considered to be a risk factor for the development of various CNS disorders including post-traumatic epilepsy (PTE) (Karlander et al., 2021) and neurodegenerative diseases, where neuroinflammation, astrocyte activation or cerebrovascular dysfunction represent important factors contributing to the progression of the disease (Jafari et al., 2013; Tjalkens et al., 2017; Ramos-Cejudo et al., 2018; Delic et al., 2020; Brett et al., 2022; Figure 9). Interestingly, rmTBI increases the glial response which may elevate the risk of delayed disorders (Cherry et al., 2016). Indeed, studies on veterans or athletes in contact sports showed a significantly increased risk of developing sleep disorders (Leng et al., 2021; Paredes et al., 2021), neurodegenerative diseases or motor and/or cognitive dysfunction compared to the rest of the population (McKee et al., 2014).
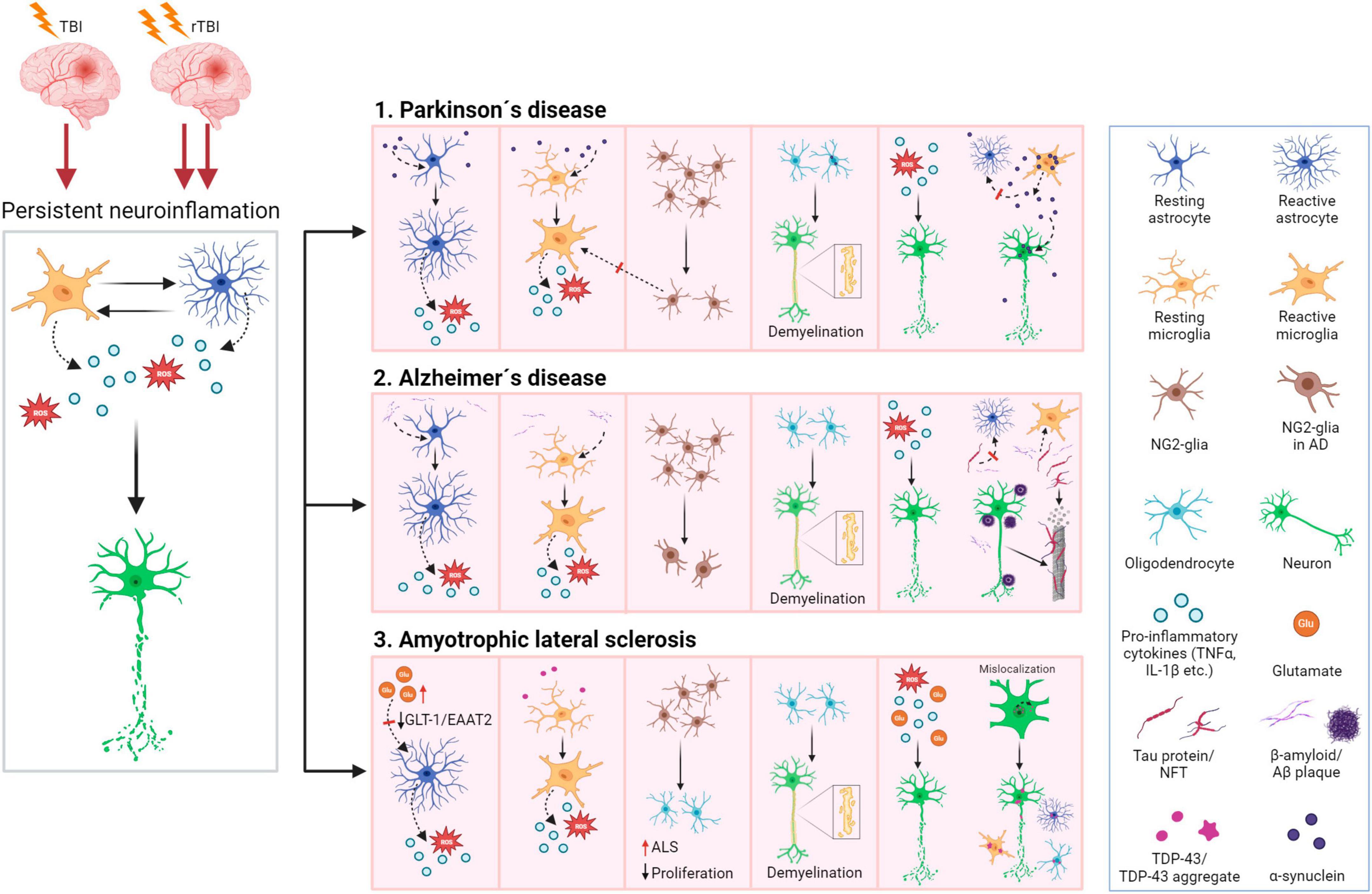
Figure 9. Traumatic brain injury as a risk factor contributing to neurodegenerative disease development and progression. Left: Persistent neuroinflammation evoked by TBI induce degenerative processes in neurons leading to their damage. Moreover, repetitive TBI has a cumulative effect on glial response which may exacerbate these processes. Right: Neurodegeneration itself induces glial activation and neuroinflammation, creating a vitious circle further progressing the disease. 1. Alzheimer’s disease (AD): astrocytes and microglia are activated by Aβ, and both release pro-inflammatory cytokines and ROS. Astrocytes and microglia are involved in Aβ/tau clearance, but astrocytic clearance is impaired due to loss of AQP4 polarity in the later stages of AD. The number of NG2-glia decreases, and the damage of oligodendrocytes causes demyelination of axons. 2. Parkinson’s disease (PD): microglia and astrocytes are activated by α-syn, and produce pro-inflammatory cytokines and ROS. Astrocytes and microglia can clear α-syn, but with the progression of PD, astrocyte capacity to internalize α-syn decreases, and microglia release the excess α-syn, causing more damage to the neurons. The number of NG2-glia decreases, which exacerbates neuroinflammation due to decreased suppression of microglial activation. Aggregation of α-syn in oligodendrocytes contributes to demyelination. 3. Amyotrophic lateral sclerosis (ALS): both microglia and astrocytes are activated and produce pro-inflammatory cytokines and ROS. Loss of astrocytic glutamate transports GLT-1/EAAT2 contributes to glutamate excitotoxicity. NG2-glia proliferation slows down with ALS progression and loss of oligodendrocytes induces demyelination. Additionally, TDP-43 aggregates can be found in microglia, astrocytes, and oligodendrocytes and further impair RNA stability and metabolism in the affected cells. For further details see the main text. Aβ, β-amyloid; α-syn, α-synuclein; TDP-43, transactive response [TAR] DNA binding protein 43; ROS, reactive oxygen species. Created with BioRender.com.
8.1 Post-traumatic epilepsy
The link between TBI and PTE is already generally acknowledged. The chance of PTE occurrence increases with the increasing severity of injury, and the probability differs based on the type of injury, e.g., diffuse TBI (8.1%) represents a lower risk of epilepsy than focal cerebral TBI (12.9%) (Karlander et al., 2021). First seizures can occur within the first week after TBI, late epileptic seizures after weeks or months following TBI. The exact causal link between TBI and PTE development remains to be fully elucidated, but the main focus is on BBB disruption and excitotoxicity, metabolic changes, oxidative stress, and particularly on neuroinflammation (Mukherjee et al., 2020; Sharma et al., 2021). TBI is also a common predisposition to dissociative seizures, their co-occurrence being between 16 and 83% (Popkirov et al., 2018). Dissociative seizures are known as psychogenic non-epileptic seizures (PNES), or functional seizures (FS), they resemble epileptic seizures but are induced by psychological distress. However, the pathophysiology of dissociative seizures is still poorly understood (Asadi-Pooya and Farazdaghi, 2021).
8.2 Neurodegeneration-associated diseases
In general, TBI and neurodegenerative diseases share similar pathogenetic mechanisms, such as neuroinflammation, disrupted glutamate clearance, or mitochondrial dysfunction and production of ROS (You et al., 2023; Figure 9). Glia itself become reactive due to the neurodegeneration–reactive gliosis can be triggered by events occurring during neurodegenerative diseases, such as accumulation of β-amyloid (Aβ) in the case of AD, or cellular changes in neurons and glia during Amyotrophic lateral sclerosis (ALS) (Burda and Sofroniew, 2014). In these diseases, glia cells often play a dual role–while neuroinflammation or reversed glutamate transport can promote neurodegenerative processes, other functions of activated glia, including Aβ clearance in AD, or the production of anti-inflammatory and neurotrophic factors in ALS, can contribute to the protection of brain tissue (Lee and Landreth, 2010; Jantti et al., 2022; You et al., 2023). The relationship to TBI and the most frequent neurodegenerative diseases as well as the role of reactive glia in their pathogenesis are discussed below.
Parkinson’s disease (PD) is a degenerative disorder of the CNS, slowly deteriorating both motor and non-motor (e.g., cognitive or gastrointestinal) functions. A characteristic of this disease is aberrant aggregation of α-syn that disturbs dopaminergic transmission. Part of the pathology is played by neuroinflammation—aggregation of α-syn serves as stimuli for microglial activation and immune response followed by neural degeneration (Calabresi et al., 2023). Despite PD being considered as a disease of the aged brain, in cases of certain professional sportsmen, including boxers or football players, or in the case of military veterans, PD-like symptoms were also found in younger patients. Based on population-based studies, TBI is considered to be one of the risk factors for PD (Jafari et al., 2013; Camacho-Soto et al., 2017). In military veterans, the increased risk of PD following TBI was 56% (Gardner et al., 2018). However, there are too many factors, such as genetic predisposition, environment, or lifestyle that further complicate PD research within a population. To overcome some of these limitations by securing similar conditions, some studies use twins in their research. These studies in veterans from the second world war found that a person after TBI will more likely manifest a cognitive decline (Chanti-Ketterl et al., 2023) and/or develop PD in comparison with his healthy twin sibling (Goldman et al., 2006). Interestingly, White et al. (2020) suggested, that comorbidity of TBI and post-traumatic stress disorder (PTSD) further increase the risk of PD in military veterans (White et al., 2020). However, these findings need to be further confirmed by other independent studies.
Despite many population-based studies, the exact association between rTBI and an increased risk of PD is not clear. Nevertheless, due to the strong involvement of glial cells in the pathogenesis of PD, it is highly probable that shifts in receptor expression and their functions in reactive glia may be an important factor initiating or contributing to PD. Astrocytes participate in α-syn and debris clearance, but this function is impaired in PD. Additionally, α-syn activates astrocytes in the later stages of PD, which start to release pro-inflammatory factors (MHCII or IL-1β). Microglia, activated by α-syn through TLRs, release pro-inflammatory cytokines that consequently promote neuronal death. Together with astrocytes, microglia contribute also to α-syn clearance in PD, but this process inhibits microglial autophagy and α-syn begins to accumulate in the cytoplasm. The excess α-syn is then released, further exacerbating the tissue damage (Zhang et al., 2022b). There is not much information about the role of NG2-glia in PD, but in the rat model of PD, the density of NG2 cells decreased in the striatum (Nascimento et al., 2023). NG2-glia regulate neuroinflammation by suppressing microglial activation, therefore a decrease in the number of NG2-glia can lead to an exacerbated immune response (Zhang et al., 2019). Oligodendrocytes may also contain aggregates of α-syn, which is linked to myelin loss (Zhang et al., 2022b). Importantly, the glial activity and response in the PD is region-dependent (Basurco et al., 2023).
Alzheimer’s disease (AD) is the most common type of dementia, characterized by memory loss, ability to learn, impaired thinking and communication. According to the World Health Organization (WHO), AD represents up to 70% of all dementia cases.1 AD is connected with the accumulation of Aβ, which form plaques, the presence of intracellular neurofibrillary tangles (NFTs) consisting of hyperphosphorylated tau proteins, and the degeneration of cholinergic neurons. Neuroinflammation exacerbates both Aβ and tau pathologies (Kinney et al., 2018). Population-based studies have shown, that people after TBI are more likely to develop AD than people with no TBI history (Li et al., 2017; Gu et al., 2022; Mielke et al., 2022); the most likely connective factor representing the reactive pro-inflammatory micro- and astroglia in post-traumatic tissue with aberrant clearance function.
Glial cells are activated in different stages of AD, with microglia being activated first, during the period of subjective cognitive decline, and astrocytes in later stages (Nordengen et al., 2019). Astrocytes are activated by Aβ, along with released microglial inflammatory cytokines. AQP4 is thought to be important for the Aβ/tau protein clearance of interstitial solutes by astrocytes. In human patients with AD, AQP4 changes localization in comparison to healthy controls (Simon et al., 2022). In later stages of AD, the clearance is impaired due to the loss of AQP4 polarity in the astrocyte endfeet (Zeppenfeld et al., 2017; Simon et al., 2022; Pedersen et al., 2023). Aβ works as a stimulus for microglial activation and simultaneously, microglia can clear out both fibrillar and soluble forms of Aβ (Kim et al., 2018). Similarly to PD, long-term exposure of microglia to Aβ disrupts the microglial autophagy, triggers mitochondrial dysfunction, ROS production, and release of pro-inflammatory cytokines, which further supports AD progression (Litwiniuk et al., 2023). Additionally, hyperphosphorylation of tau proteins can be induced by microglial activation. Still, activated microglia can regulate the number of tau proteins through phagocytosis or by releasing tau proteins from microglia in exosomes (Uddin and Lim, 2022). NG2-glia change morphology in AD and their number is decreased in human AD (e.g., in animal APP/PS1 model of AD, NG2-glia proliferate) (Nielsen et al., 2013; Dong et al., 2018). In the early stages of AD, oligodendrocytes are already damaged, followed by myelin loss (Butt et al., 2019).
Amyotrophic lateral sclerosis (ALS) is a neurodegenerative disease resulting in muscle weakness, paralysis, and death, due to the degeneration of motor neurons. We can distinguish two types of ALS: familiar (approximately 10% of cases) and sporadic (You et al., 2023). To date, several contributors to neuronal damage have been identified, such as genetic mutations (SOD1, FUS, or C9ORF72) disregulating RNA metabolism, increasing oxidative stress or generating protein aggregates, including TDP-43 (transactive response [TAR] DNA binding protein 43), that can be present in neuronal/glial cytoplasm (Filipi et al., 2020). TDP-43 was shown to have multiple functions in transcriptional repression, pre-mRNA splicing and translational regulation (Sephton et al., 2011) and besides ALS tissue can be detected also in patients with chronic traumatic encephalopathy (CTE) associated with rmTBI (de Boer et al., 2020; Murray et al., 2022).
In comparison to other neurodegenerative diseases, such as AD or PD, there is not sufficient evidence, that TBI is a risk factor for ALS. However, it is believed, that reactive gliosis and inflammation (both long-term processes in TBI patients, as well as in aged brains) contribute to ALS progression (Maragakis and Rothstein, 2006). There are some population-based studies (Lian et al., 2019; Tsai et al., 2019; Filipi et al., 2020), but they have limitations (e.g,. small sample size). A further complication is the unavailability of models which would fully capture the complexity of ALS (Fisher et al., 2023). For example, one of the most frequent mutations in ALS is in Cu/Zn Superoxide dismutase 1 (SOD1) and rodents carrying the SOD1 (G93A) mutation is a commonly used model of this disease. In addition to other problems, including a decrease in the copy number of the mutation in chromosome 21 affecting ALS severity (Bonifacino et al., 2021), a recent study showed minimal disease-related changes in microglia and oligodendrocytes in SOD1 (G93A) mouse cortex during the end-stage of ALS, which contrasts with findings in patients (Filipi et al., 2023).
Amyotrophic lateral sclerosis research is primarily focused on motor neurons, but in recent years, the focus of researchers was shifted to glial cells, such as microglia and astrocytes (Neusch et al., 2007; Calafatti et al., 2023; You et al., 2023). Astrocytes in the ALS brain become reactive, they contribute to neuronal damage due to glutamate excitotoxicity caused by a loss of GLT-1/EEAT2, dysregulated release of neurotrophic factors, production of pro-inflammatory cytokines, or ROS production. Astrocytes can also contain cytoplasmic stress granules consisting of mutated TDP-43, ubiquitin, and similarly as in PD, α-syn. Microglia are activated, release pro-inflammatory cytokines (e.g., TNF-α, IL-1β) and ROS. The microglial immune response can be evoked by TDP-43 aggregates; microglia can also be more sensitive to inflammatory stimuli. TDP-43 aggregates in microglial cytoplasm in ALS patients. Very little is known about the role of NG2-glia but their proliferation and maturation into oligodendrocytes slows down with the ALS progression. Oligodendrocytes are damaged, which evokes axon demyelination of motor neurons. Similarly to astrocytes and microglia, oligodendrocytes can also contain TDP-43 aggregates (Philips and Rothstein, 2014; Filipi et al., 2020).
8.3 Motor and cognitive dysfunction
Traumatic brain injury-induced neuropathology significantly influences both motor and cognitive dysfunction (Gorgoraptis et al., 2019; Corrigan et al., 2023). Behavioral outcomes in various animal models that have been established to replicate diverse clinical TBI pathologies, can consequently vary. Comparing and contrasting results from different behavioral tasks within (e.g., mild vs. moderate vs. severe; acute vs. subacute vs. chronic) and between (e.g., diffuse, focal, mixed, blast) the models and pathologies is a crucial aspect in utilizing various behavioral models of TBI for a deeper understanding of its diverse aspects and subsequently for therapeutic applications (Shultz et al., 2020). Additionally, the choice of experimental species and strains is an important factor, due to differences in learning abilities, memory, attention, or responsiveness to stress and drug treatments (Fujimoto et al., 2004).
Understanding the causal mechanisms behind behavioral changes in TBI, whether in patients or animal models, is limited. Yet evidence suggests that animal models can replicate pathologies and behavioral abnormalities seen in human TBI. Here, we outline some of the common behavioral tests used for motor and cognitive assessments and model-related findings. It is important to note that protocols used in individual studies differ (even the order and number of tests can have a huge impact on the outcome), therefore the issue of standardization should be considered. Detailed information about specific behavioral tests and post-traumatic motor and cognitive deficits following different TBI models can be found in comprehensive reviews (Fujimoto et al., 2004; Shultz et al., 2020).
Assessment of motor functions: motor coordination tests are valuable tools for studying the effects of brain injuries on motor skills, balance and physical condition. Following TBI, motor deficits can be caused by injury-related disruption of the complex motor pathways between the cortex, sensorimotor cortex, subcortical nuclei cerebellum and brainstem (Fujimoto et al., 2004). The Rotarod test is commonly used to assess motor coordination and balance in rodents. In this test, the CCI model (moderate, rat) seems to impact the animal performance the most, as injury effects were detectable even 11 weeks post-TBI (Lindner et al., 1998). In comparison, midline FPI (moderate, rat) showed that injury affects performance for up to 5 days (Hamm et al., 1994). The Beam balance test (BBT) provides valuable insights into the impact of TBI on an animal’s ability to maintain stability and coordinate movements, which are crucial aspects of motor function. Interestingly, in this type of test, the longest impact post-TBI was found in lateral FPI (moderate, rat, the effect of injury lasted up to 1 week) (Long et al., 1996). Midline FPI (moderate, rat) affected the test performance for up to 4 days (Dixon et al., 1987) and CCI (moderate, rat) for up to 3 days (Hamm et al., 1992). The Open field test evaluates general locomotor activity, exploration, and anxiety-related behaviors (Fujimoto et al., 2004; McDonald et al., 2021). After CCI (mouse model), severe injury resulted to reduced locomotor activity, but not mild or moderate injury (Dixon et al., 1999; Zhao et al., 2012).
Assessment of neurocognitive outcome: these tests evaluate learning ability and memory. A widely used behavioral assay in TBI research is the Morris Water Maze (MWM) test to assess spatial learning and memory in rodents. The MWM is particularly valuable for investigating cognitive and learning deficits, working memory and retrograde/anterograde amnesia associated with TBI (Morris, 1984; Washington et al., 2012; Tucker et al., 2018). Spatial learning was impaired in the rat CCI model (moderate for more than 1 year post-TBI) (Dixon et al., 1999), and in midline FPI (moderate, rat) for up to 18 days (Yamaki et al., 1998). Mild lateral FPI (rat) did not affect learning ability, a moderate one (rat) showed an effect for up to 8 weeks (Sanders et al., 1999) and severe lateral FPI (rat) caused learning deficit for more than 1 year (Pierce et al., 1998). Effects are also severity-dependent (the mouse CCI model), with severe TBI having the worst outcome (Zhao et al., 2012). The Barnes Maze represents an alternative paradigm in assessing cognitive functions and allows researchers to explore the TBI impacts on a spatial navigation and memory retention in a different context than MWM (Barnes, 1979; Rosenfeld and Ferguson, 2014). In Barnes maze, rats after moderate lateral FPI developed different search patterns compared to controls (Fedor et al., 2010). Mild CCI (mouse) shows impairment after 3 months post-injury (Taib et al., 2017). Some studies reported even sex differences, where male mice displayed unique spatial memory deficits after moderate lateral FPI, but not females (Fitzgerald et al., 2022). The Novel Object Recognition (NOR) in TBI research serves as a behavioral assay that specifically targets declarative memory, providing valuable information on the cognitive consequences of TBI in rodent models. In the CCI mouse model, mild injury did not show any differences compared to control, but moderate and severe injury led to learning and memory impairment (Zhao et al., 2012; Fidan et al., 2016).
9 The role of senescent glial cells in traumatic brain injury and neurodegeneration
Senescent cells, characterized by damage and resistance to apoptosis, actively contribute to biochemical changes associated with neurodegeneration. These alterations include abnormal protein aggregation, metabolic shifts, autophagy deficits, mitochondrial dysfunction, oxidative stress, and impaired neurogenesis (Musi et al., 2018; Chapman et al., 2019; Chini et al., 2019). Senescent cells, influenced by the cell type and initiating stimulus, typically undergo a phenotypic change, with around 30–70% developing a senescence-associated secretory phenotype (SASP). SASP involves elevated, cell-dependent secretion of inflammatory cytokines such as IL-1β, IL-6, and TNF-α, chemokines, matrix metalloproteinases (MMPs), as well as proteases and growth factors. All these alterations contribute significantly to chronic inflammation and inflict damage on neighboring cells (Tchkonia et al., 2013; Baker and Petersen, 2018). Unlike neurons, glial cells exhibit a higher propensity for senescence (Salminen et al., 2011; Fielder et al., 2017; Cohen and Torres, 2019). Both astrocytes and microglia can undergo senescence, which contribute to neurodegeneration during aging or brain pathologies such as TBI, AD, and PD (Salminen et al., 2011; Baker and Petersen, 2018; Kritsilis et al., 2018).
In the context of TBI, a study using CCI in both young and aged mice revealed increased DNA damage and the expression of senescent markers in microglia within the injured brain 72 h post-injury (Ritzel et al., 2019). Senescence-associated beta-galactosidase (SA-β-Gal) positive cells, a specific marker of cellular senescence, persisted in the ipsilateral hemisphere for 1 month following TBI (Tominaga et al., 2019; Schwab et al., 2021). Elevated SA-β-Gal was observed throughout the cortex, hippocampus, and thalamus at 1 day and 1 month following a single or repeated moderate bTBI in rats (Arun et al., 2020). Moreover, SA-β-gal activity remained elevated for over 2 weeks post-TBI, accompanied by an increased expression of p16INK4a, a cyclin-dependent kinase inhibitor associated with senescence, specifically noted in astrocytes following TBI (LaPak and Burd, 2014; Tominaga et al., 2019).
Additionally, postmortem brains of professional athletes with multiple mTBI similarly showed increased DNA damage, senescence marker expression, and the presence of SASP factors (Schwab et al., 2019). Moreover, rmTBI in mice exhibited elevated DNA damage markers at 24 h and increased expression of p21, p53, and SASP, particularly IL-1β, in the injured cortex 7 dpi (Schwab et al., 2021). However, the administration of ABT263, the senolytic drug, 1 week after rTBI significantly enhanced performance in the MWM (Schwab et al., 2022).
Several important conclusions emerged from a study by Wang J. et al. (2023). Following TBI, their findings supported the long-term neurodegeneration in the cortex, hippocampus, dentate gyrus, and thalamus, associated with a strong neuroinflammatory response, white matter damage, and a significant deficit in cognitive function and depressive-like behavior (Wang J. et al., 2023). These results are in line with human research showing that white matter damage and neuroinflammatory events in the brain can last for many years following TBI (Johnson et al., 2013b). Furthermore, nearly 50% of hospitalized TBI patients have impairments from their injuries after a year, including depression, anxiety, and cognitive impairment (Johnson et al., 2013b). Secondly, an investigation by Wang J. et al. (2023) revealed widespread occurrence of senescent cells in various brain regions persisting for weeks to several months following TBI. Through double immunohistochemistry, it was observed that these senescent cells included both astrocytes and microglia. This observation aligns with prior studies that identified senescent astrocytes and microglia in the injured cortex shortly after TBI (Ritzel et al., 2019; Tominaga et al., 2019). Recent findings indicate that senescent astrocytes, displaying reduced neuroprotective functions due to glutamate transporter down-regulation, may contribute to prolonged neurodegeneration post-TBI (Limbad et al., 2020).
Senolytic therapy, eliminating senescent astrocytes and microglia, resulted in reduced neurodegeneration and increased neuron numbers in multiple brain regions in animals with TBI (Wang J. et al., 2023). Senescent cells also release SASP factors, promoting chronic neuroinflammation and causing damage to neighboring cells. Wang J. et al. (2023) also showed that proinflammatory SASP factors, IL-1β and IL-6 were significantly elevated in the ipsilateral cortex at 4 months post-TBI and a senolytic therapy with dasatinib and quercetin (D + Q) treatment markedly reduced this increase, indicating a substantial reduction in the long-term inflammatory burden (Wang J. et al., 2023). Persistent inflammation after TBI is linked to unfavorable outcomes (Kumar et al., 2015). D + Q therapy not only lowered the senescent cell burden but also mitigated chronic neuroinflammation, aligning with the potential benefits of targeting IL-1β and IL-6, known to improve cognitive function after TBI (Clausen et al., 2011; Flygt et al., 2018). The potential involvement of senescent cells in prolonged functional deterioration post-TBI is underscored by the effectiveness of delayed, intermittent D + Q senolytic treatment initiated 1 month after TBI. This intervention notably eradicated senescent cells and resulted in a significant amelioration of both cognitive impairment and depressive-like behavior in TBI mice at the 4-month post-injury interval. This extension of the therapeutic window, beyond the conventional acute treatment timeframe, holds clinical significance by offering potential interventions for patients well into the chronic phase of TBI, where therapeutic options are currently scarce (Wang J. et al., 2023). For further information about senolytic and senomorphic therapy see chapter “TBI diagnostics and treatment.”
10 Traumatic brain injury in military and veterans and special features of mild blast injury
Soldiers and war veterans are a group of TBI patients providing valuable data for population-based studies of TBI—their medical history is usually well documented while being at high risk of TBI, such as BI, bullet penetration, falls, or head impacts. According to the Defense and Veterans Brain Injury Center (DVBIC), approximately 82% of reported TBI sustained by members of the U.S. Armed Forces between 2000 and 2023 was classified as mTBI.2 TBI affecting military personnel was linked or correlated with visual dysfunctions, sleep disorders, development of psychiatric sequelae including depression and PTSD, and higher risk of neurodegenerative disorders (McKee and Robinson, 2014; Hussain et al., 2021; Leng et al., 2021; Kong et al., 2022).
The most common TBI affecting soldiers is mild bTBI after exposure to explosives and their overpressure wave (McKee and Robinson, 2014; Hernandez et al., 2018). However, soldiers are not the only ones affected by bTBI, the civilian population can suffer bTBI due to terrorist bomb attacks or presence in an active war zone. Overpressure waves cause compression of brain tissue as well as the movement of the brain in the skull, causing more damage by impact (Bryden et al., 2019). These events evoke hemorrhaging, edema, axonal injury, or neuroinflammation (McKee and Robinson, 2014).
Blast TBI exhibit common as well as unique features compared to concussive-type TBI, where there is evidence of astrogliosis, maladaptive inflammatory response and BBB breakdown (Rubovitch et al., 2011; Wilk et al., 2012). Close analysis has shown the induction of microvascular and axonal damage and neuroinflammation in the cortex, striatum and hippocampus. These changes correlate with deficits in synaptic function and hippocampal plasticity. Biochemical analysis revealed overactivated calpain, indicating disrupted Ca2+ homeostasis after mild bTBI (Hernandez et al., 2018).
Logsdon et al. (2018), in agreement with other studies confirmed that a single blast exposure rapidly and temporarily disrupts the BBB, allowing abnormal entry of sucrose and albumin traces into the various brain regions. BBB integrity is restored for albumin but not sucrose by 24 h (Logsdon et al., 2014, 2018; Huber et al., 2016; Mishra et al., 2016). Following two consecutive mild blast exposures cause the BBB to reopen to albumin at 72 h. Tight junction disintegration aligns with the second phase of BBB disruption. These results indicate immediate and prolonged brain permeability to small molecules, coupled with delayed, region-specific permeability to larger molecules (Goodrich et al., 2016). Despite the prevalent role of astrocytes in TBI, their contribution to mild bTBI remains unclear. In blast-exposed swine hippocampus, elevated expression of inflammatory proteins has been found (Goodrich et al., 2016), supporting the idea that blast-induced inflammation may involve astrocytic activation. Astrogliosis observed in animal BI and CHI models (Kernie et al., 2001) also appears in post-mortem brains of blast-exposed soldiers, particularly evident in the hippocampus. Goodrich et al. (2016) on the model of Yorkshire pigs showed activation of astrocytes 2–4 weeks post single blast exposure (Goodrich et al., 2016). In animals exposed to double or triple blasts with longer survival times (6–8 months), there is a 20–30% increase in activated astrocytes in the dentate hilus and molecular layer compared to single blast exposure. Elevated astrocyte density, noted in CA1, may respond to observed neuronal injury. In the hilus, where significant neuronal injury was observed in the single blast-exposed group, but an increased astrocyte number suggests an early involvement in the hippocampal injury process. Proteomic analysis confirms a rapid elevation in GFAP levels, supporting early astrocyte activation post-blast (Ahmed et al., 2012). On the other hand, microglial activation is not so widespread (Goodrich et al., 2016). Activated microglia, mainly in white matter regions like the corpus callosum, were prominent in animals with double and triple blast exposure. However, despite their presence, there was no detectable axonal degeneration observed through Aβ immunostaining in the same areas, while in post-mortem brains of blast-exposed soldiers, activated microglia were associated with patches of Aβ injured axons. The precise role of activated microglia in blast mTBI in white matter tracts thus remains unclear. More findings regarding glia in rodent model of repetitive bTBI are available in Table 1.
Recent studies delve deeper into the impact of mild blasts on cognitive, metabolic, and behavioral functions. Kumari et al. (2023) specifically explored hippocampal metabolic changes after mild, moderate and rmTBI in rats (Kumari et al., 2023). Significant alterations in N-Acetylaspartic acid (NAA), acetate, PC/choline, phosphoethanolamine (PE), ethanolamine, glutamate, and creatine concentrations were noted compared to Sham only in the rmTBI group. These metabolic shifts may affect biochemical processes and metabolic-dependent mechanisms such as the epigenome, highlighting the intricate link between metabolism and epigenetics (Kumari et al., 2023). A study by Kumari et al. (2023) also showed an Alanine (Ala) reduction in the hippocampus 24 h post-mild, moderate, and repeated bTBI, which aligns with another study by Norris et al. (2023). This study, quantifying acute changes after bTBI in the metabolism of free amino acids as molecular precursors, neurotransmitters and metabolic regulators in rats, found alterations in Arg and Ala pathways linked to oxidative stress following bTBI (Norris et al., 2023). Decreased Arg may elevate oxidative stress via NOS and contribute to pro-inflammatory cascades (Mader and Czorlich, 2022). Administering Arg post-TBI showed neuroprotective effects, while the NOS inhibitor L-NAME improved the BBB function and sensorimotor impairment post-blast exposure (Logsdon et al., 2020). Modulating the NO/Arg pathway may thus have therapeutic potential, warranting further investigation into acute Arg changes in various tissues.
Animal models of bTBI may be restricted due to interspecies variations in the neuropathophysiology between the animal and human brain. Using 2D or 3D human cell cultures for in vitro modeling also has its limitations (Fang and Eglen, 2017; Qian et al., 2019). Snapper et al. (2023) introduced a new in vitro model based on a bioengineered 3D brain-like culture system that can mimic the human pathology fairly well (Snapper et al., 2023). Using this novel bTBI model they revealed increased axonal varicosities following primary bTBI, persisting for a week with a peak at 72 h. These varicosities, indicative of traumatic axonal injury, show the accumulation of amyloid precursor protein (APP), suggesting a blast-induced deficit in axonal transport. The study also found elevated NF-H and UCH-L1 (Ubiquitin carboxy-terminal hydrolase L1, known neuronal damage markers) at 6 h, S100B (astrocytic marker) at 24 and 48 h post-blast, indicating sequential damage. GFAP didn’t increase, possibly due to elevated pre-injury levels. This novel in vitro culture system without a BBB may allow controlled screening for new biomarkers that are pivotal in diagnose blast-induced mTBI (Snapper et al., 2023).
11 Factors affecting traumatic brain injury outcome in experiments
Traumatic brain injury is a complex process and due to its intricacy, creating a model that closely mimics human pathology is difficult. As many previous studies have shown, there are differences within TBI models, including their impact on glial cells and different reported results depending on the severity and location of injury as well as on model accuracy, reproducibility and repeatability. However, the type of TBI model is not the only aspect which is necessary to be considered—factors, such as age and sex of laboratory animals are also essential for the glial response. In this section, we will summarize some of these factors and their impact on glial cells.
11.1 Age and the impact of aging on traumatic brain injury
The age of an experimental animal is a crucial concern when designing experiments. Compared to the youthful brain, glial cells in the aged brain exhibit changes in shape and protein expression (Alibhai et al., 2018). Based on the genomic analysis of ischemic tissue, Androvic et al. (2020) unveiled a significant overlap in the inflammatory response, cell-cell interactions, and cell cycle progression between young adult, and aged animals (Androvic et al., 2020). Animals commonly used in research experiments are frequently in the prime of their productive adult lives. Nevertheless, it is important to note that the aging brain is more susceptible, and the elderly are at an elevated risk of TBI due to pre-existing medical conditions, a heightened likelihood of falls, natural anatomical changes, and other factors, as discussed by (Yee and Jain, 2023). Another crucial factor is the fluctuations in hormonal levels during aging, as they have a significant impact on the glial response (Arevalo et al., 2010). Since the female hormone cycle and/or menopause may have an impact on TBI outcomes, the inclusion of females in studies of the aging effect on TBI results is crucial.
During aging, an increased expression was detected in some proteins that were shown to be involved in the tissue response to TBI, such as GFAP, Vim, and AQP4 (Nichols et al., 1993; Porchet et al., 2003). Moreover, astrocytic subpopulations that differ from those in young tissue in morphology, function, or membrane properties, were identified during aging (VanGuilder et al., 2011; Matias et al., 2019; Verkerke et al., 2021). Based on RNA sequencing, Clarke et al. (2018) found that due to aging, astrocytes change their transcriptome and become reactive in a region-dependent manner. In response to the neuroinflammation promoted by microglia, the number of reactive astrocytes is significantly higher in aged tissue than in young brains (Clarke et al., 2018). During aging, microglia undergo morphological and functional alterations and subsequently become senescent/dystrophic (Streit et al., 2004). Aging is also associated with microglial priming which results in higher sensitivity to stimuli and exaggerated microglial responses (Henry et al., 2009).
It was demonstrated that a cognitive decline in aged patients, besides other morphological brain changes, is also associated with an overall loss of white matter (Chopra et al., 2018). The most impacted structures are the anterior-posterior gradient and the prefrontal cortex, and the anterior corpus callosum (Head et al., 2004; Bennett et al., 2010). The white matter damage seems to be promoted by persistent NF-κB signaling, which is a pathway also inducing the senescence of oligodendrocytes (Schlett et al., 2023). Waller et al. (2019) investigated the microglial populations present in deep subcortical lesions (DSCLs) of the brain, which are present in approximately 60% of the population over 65 years. DSCL is a white matter lesion linked with myelin loss, BBB dysfunction, and gliosis. In human autopsy tissue, a significant decrease of microglia marker Iba1 and an increase of CD68 expressions in the DSCL group as well as shifted morphology of CD68-positive (CD68+) cells from ramified to more rounded cells were found. Of note, some CD68+ cells did not express Iba1, indicating the presence of microglial subpopulations. The authors also found that myelin integrity in DSCL was reduced. In contrast, another type of white matter lesions presented in aged tissue, periventricular lesions (PVLs), contained more activated microglia with a ramified phenotype. In this phenotype, a higher MHCII expression was detected, as well as increased expression of B7-2 and CD40 (both co-stimulatory molecules of MHCII) than in the DSCL microglia (Waller et al., 2019). PVL is also a more microglia proliferation supporting environment than DSCL (Simpson et al., 2007). Additionally, age-related impairment of progenitor recruitment and the consequent differentiation into oligodendrocytes causes a decrease in remyelination efficiency in the elderly (Sim et al., 2002).
Following CCI, age-related significant differences in microglial activation were detected (Kumar et al., 2013). This study showed increased expression levels of genes such as IL-1β, CD86, and TNF-α, upregulation of MHCII mRNA and upregulated expression levels of MHCII, as well as a higher number of activated microglia in the cortex, hippocampus, and thalamus. Moreover, 2 different microglial phenotypes were observed. The authors also revealed that the volume of lesions in the aged brain is higher, which is combined with a significantly increased neuronal loss in the aged group, in comparison with the young group (Kumar et al., 2013). Compared to young adult tissue, the astroglial and microglial reaction after CCI was delayed in the hippocampus of the aged brain; a similar response of astrocytes was detected in the cortex (Sandhir et al., 2008; Early et al., 2020). Furthermore, the hippocampus appeared to be more vulnerable to TBI-induced morphological changes of reactive astrocytes (Early et al., 2020).
Besides astrocytes and microglia, the function of other glial cells during aging is also altered. NG2 cells in gray matter differentiate into oligodendrocytes faster in young mice than in older mice (Hill et al., 2014). Viney et al. (2022) used the THY-Tau22 tauopathy mouse model to show the spread of phosphorylated tau from neurons to oligodendrocytes in the hippocampus of aged mice (Viney et al., 2022).
These alterations may have a great impact on tissue damage and recovery of the aged brain after TBI, as well as higher levels of neurodegeneration in the elderly. Unfortunately, TBI models do not reproduce responses in aging brains in full detail, since aged experimental animals lack the comorbidities usually found in the elderly, such as diabetes, hypertension, and cardiovascular diseases.
11.2 Sex differences
Based on epidemiological studies, the incidence of TBI is higher in men than in women (Faul and Coronado, 2015; Peeters et al., 2015; Capizzi et al., 2020; Brazinova et al., 2021). Unfortunately, the study sample based on sex is not usually equally distributed (Bodnar et al., 2019; Gupte et al., 2019; Biegon, 2021), therefore, the results of some studies may be misinterpreted. The levels of hormones progesterone and estrogens, especially estradiol, seem to be an important factor in TBI outcome (Roof et al., 1994; Bramlett and Dietrich, 2001). Specifically, 17β-estradiol modulates the adult microglial phenotype and decreases neuroinflammation after TBI (Wang et al., 2021). The ability of these hormones is in tight cooperation with astrocytes and microglia (Johann and Beyer, 2013). Menzies et al. (2011) demonstrated that progesterone can shift the activation type of the macrophages by the selective regulation of macrophage gene expression (Menzies et al., 2011).
In some studies, with a focus on the sex of the experimental animals, differences in glial cell response were observed (Acaz-Fonseca et al., 2015; Villapol et al., 2017). For example, in the CCI model, males displayed more rapid astrogliosis and a larger increase in lesion volume in comparison to females (Villapol et al., 2017). Moreover, the study also revealed differences in microglial activation, morphology, and phenotype diversity, as well as in the inflammatory response and mRNA levels, with a greater extent of reactive microgliosis found in male rather than female tissue post-TBI. The differences in neuroinflammatory responses were also observed in the cortical stab injury model. Interestingly, the number of neurons in the lesion site was higher in males, but no difference in the number of astrocytes between males and females was detected. Male samples showed a higher percentage of an astrocytic subpopulation expressing chemokine (C-C motif) ligand 2, which is an important mediator of neuroinflammation (Acaz-Fonseca et al., 2015). In the WD model, only mild differences in glial response between sexes, even in rTBI were observed (Shandra et al., 2019). Males and females also exhibited different results in behavioral testing post-TBI in the lateral FPI model, with females expressing greater sensitivity to sensory stimuli, such as light or noise, than males (Hoffman et al., 2020).
11.3 Chemicals used for anesthesia
Anesthesia is widely used in experiments to prevent animal suffering during the induction of TBI. In general, anesthesia, especially long-term exposure can result in brain damage, specifically the induction of the mitochondrial apoptosis, which evokes neuronal death (Mesa Suarez et al., 2016; Wu et al., 2019). Furthermore, the following studies revealed the effect of different chemicals used for anesthesia on the reaction of various glial cells. Drugs used for induction of general anesthesia, such as isoflurane, suppress LPS-induced expression of microglial pro-inflammatory cytokine IL-1β in mouse brains (Tanaka et al., 2013). On the other hand, prolonged anesthesia using sevoflurane induces neuroinflammation by triggering the NF-κB pathway, activates microglia, and alters their morphology in the hippocampus of aged rats (Xu et al., 2023). According to Brambrink et al. (2012), exposure to isoflurane for 5 h causes apoptosis of oligodendrocytes (estimated at 6.3% of the total oligodendrocyte population) in neonatal primate brains (Brambrink et al., 2012). Isoflurane also inhibits Na+/K+-ATPase, specifically causing a decrease in the activity of astrocytic and neural isoform of Na+/K+-ATPase (approximately 25% decrease after 1-h exposure) and therefore disrupting ion homeostasis (Reiffurth et al., 2023). Sun et al. (2019) compared isoflurane and ketamine anesthesia using a mouse model with a cranial window. Isoflurane caused significantly elongated microglial processes in both acute (1–2 days post-surgery) and chronic experiments (4 months after surgery) and a faster reaction of microglia to photodamage in chronic trials. Ketamin only increased the number of microglial branching in acute experiments (Sun et al., 2019). Furthermore, isoflurane decreases the expression of α-tubulin and GFAP directly during anesthesia and even 2 days following 4 h of exposure in vitro (Culley et al., 2013).
11.4 Frequency of traumatic brain injury
Multiple mild head injuries are risks associated with military service or contact sports, such as boxing. The importance of investigating rmTBI is high, because of the link to the development of CTE or a higher risk of dementia/AD (Uryu et al., 2002; McKee et al., 2009, 2016). Cherry et al. (2016) studied the post-mortem brains of American footballers and discovered an altered microglial phenotype, and increased density of DC68+ cells. The authors then correlated the increased neuroinflammation with longer exposure to rmTBI. These results propose the theory that cumulative damage results in a stronger glial response (Cherry et al., 2016). Amplification of glial activation was also shown in the CCI-CHI model with a midline impact resulting in bilateral injury; TBI repeated after 24 h exhibited a significantly increased microglial response than single impact, but prolonging intervals between TBI to 48 h evoked similar results to the single TBI (Bolton and Saatman, 2014). Similarly, results in lateral CCI-CHI repeated after 24 h revealed that rmTBI–affected brain tissue evinced increased microglial activation in comparison to single TBI. The level of the microglial response in the observed brain regions was variable in time, with the largest microglial response to rmTBI at 4 dpi in the hippocampus, 7 dpi in the cortex, and 28 dpi in the thalamus (Shitaka et al., 2011).
Xu et al. (2016) observed activated deramified microglia (Iba1+ cells, many of them also CD68+) in the superior colliculus, corticospinal tract, corpus callosum, and part of the cerebellar white matter in rmTBI using the Marmarou WD model. Additionally, phagocytic reactive microglia colocalized with injured axons in the optic nerves, optic tract, corticospinal tract, and cerebellar lobule (Xu et al., 2016). Briggs et al. (2016) reported astrocytic and microglial reactivity in the corpus callosum and optic tract, white matter thinning of the corpus callosum, and myelin loss in the WD model of injury. Their data also suggest that rmTBI has an accumulative effect. However, their conclusion could be misleading, as instead of comparing single-impact mTBI with rmTBI, the authors only compared rmTBI animals with control animals anesthetized with isoflurane (Briggs et al., 2016). On the other hand, the population of NG2-glia in CCI rmTBI was not affected in the corpus callosum or cortex; a significant change in myelination was not detected either (Yu et al., 2017). The rmTBI also exacerbates the astroglial response in the CCI-CHI model if repeated after 24 h (Uryu et al., 2002; Bolton and Saatman, 2014). However, in the WD model of rmTBI, Shandra et al. (2019) did not observe an induction of GFAP+ astrocyte proliferation (Shandra et al., 2019). We have compiled these research findings concerning glial cells in experimental models of rmTBI, based on the results discussed in this article in Table 1.
12 Genomic methods in traumatic brain injury research
An important aspect of TBI research involves the development of novel methods, their improvement, and integration with established ones. Advanced methods have emerged in neuroscience in recent years, specifically focusing on the application of cutting-edge procedures of single-cell transcriptomics.
For single-cell transcriptomics, two basic methods are used: single-cell RNA sequencing (scRNA-seq) and single-nucleus RNA sequencing (snRNA-seq). The samples for scRNA-seq must be fresh and require tissue disintegration. Alternatively, samples for snRNA-seq can be frozen (an advantage when using samples from patients) or fixed. Additionally, snRNA-seq is more suitable for fragile cells (e.g., neurons), which are difficult to dissociate intact. However, the disadvantage of snRNA-seq is analysis predominantly focused on nuclear transcripts. Both scRNA- and snRNA-seq methods have their limits, for example they lack spatial information (Piwecka et al., 2023). To overcome the limitations and biases of sc/snRNA-seq, other studies use some of the following strategies: multimodal analysis (more than one molecular feature is analyzed, integrating data from transcriptome with epigenome or proteome from the same cell), combination with physiology or morphology analysis (incorporation with electrophysiological recordings, e.g., scRNA-seq and calcium imaging), spatial transcriptomics (RNA analysis while spatial information is preserved, mapping the positions of cells using in situ hybridization), or perturbation experiments (integration of genome editing for loss/gain functional studies) (Mayer et al., 2019; Armand et al., 2021).
scRNA-seq allows to reveal transcriptomic shifts or cell-to-cell interactions (by mapping gene co-expression). For example, in the model of lateral FPI, injury enhanced interaction between astrocytes and neurons, as well as between microglia and oligodendrocytes, while the interaction of microglia and oligodendrocytes with neurons was decreased in comparison with the controls (Arneson et al., 2018). These observations suggested TBI-dependent reorganization processes. The most enriched DEGs (differentially expressed genes) after TBI were those involved in metabolic depression and calcium/calmodulin pathway in astrocytes, inflammation in microglia, myelination and oligodendrocyte differentiation in oligodendrocytes and myelination and immune response in OPCs. The most significantly differentially expressed cell-type specific genes were p2ry12 (gene of purinergic receptor, role in microglial activation) in microglia, and Trf (gene of transferrin receptor) in oligodendrocytes (Arneson et al., 2018).
Arneson et al. (2022) identified a new treatment target, humanin and its gene mt-Rnr2, based on spatiotemporal alterations in the lateral FPI model. Expression levels of several DEGs which were elevated post-injury, decreased after humanin administration. Additionally, the treated animals showed improvement in cognitive ability. The authors also mapped TBI-evoked region-dependent changes in glial cells in the hippocampus and frontal cortex during the acute and subacute phases of TBI. In the hippocampus, the genes involved in oxidative phosphorylation and ETC were downregulated in astrocytes and oligodendrocytes (acute phase), while in microglia, TBI induced upregulation of metabolic pathways (subacute phase) (Arneson et al., 2022).
Garza et al. (2023) used snRNA-seq to analyze human samples after severe TBI. Injury causes transcriptional changes in oligodendrocytes, triggering an immune reaction and interferon response (via STAT1/2) that activates MHC-related genes. Similarly, immune processes can also be detected in OPCs. In addition, transcription of several endogenous retroviruses was activated in oligodendrocytes and OPCs, and some level of activation was detected in microglia following TBI. This retroviral activation could potentially lead to the activation of immune pathways (Garza et al., 2023).
Microglia and astrocytes are the most affected cells in the subacute phase in the lateral FPI model. During this phase, several signaling pathways were enhanced including CCL (CC chemokine ligand, indicates neuroinflammation) and non-canonical neurotrophic factor signaling. Non-canonical neurotropic factors MDK (Midkine), PTN (Pleiotrophin), and PSAP (Prosaposin) were upregulated in astrocytes and microglia. Based on in vitro testing, astrocytic expression of MDK, PTN, and PSAP is enhanced by activated microglia. LPS induction directly evoked only upregulation of PSAP in BV2 microglia (Qiu et al., 2023).
Data from sequencing can be processed into a library or interactive atlas (Network, 2021; Zhang et al., 2023). Such atlases are available, for example on the online platform Allen Brain Cell Atlas, created from scRNA-seq and spatial transcriptomic datasets from the whole mouse brain (Yao et al., 2023). Hence, these datasets and atlases present opportunities to uncover connections that were challenging to discern across a diverse array of fields. Sc/snRNA-seqs are also very useful in the investigation of brain tumors, neurodegeneration, or psychiatric disorders (Antunes and Martins-de-Souza, 2023; Pozojevic and Spielmann, 2023; Walter et al., 2023). Since even small changes in the transcriptome can be detected, it can help to identify the involvement of different cellular subtypes in diseases or outline time-dependent changes which would be otherwise undetectable. The application of single cell/single nucleus methods also have a significant impact on clinical research, showing the possibility of new treatment targets as well as tools for effective diagnostics.
13 Current challenges and future directions
The field of TBI research is highly dynamic, and presents many challenges, which can be overcome only with newly developed and improved techniques and analytic methods. Here, we list some of the most important items that should be considered in future research:
13.1 Heterogeneity of traumatic brain injury outcome in experimental models and the model limitations
Traumatic brain injury animal models are useful and still irreplaceable tools in brain injury research. However, TBI is not simple to imitate, the observations in patients are complex, and to date, no model can contain all of them. The key to success is to carefully design the experiment and determine which aspect of secondary injury we would like to focus on. The absence of comorbidities, which are present in older patients, the impact of hormones and their fluctuation, the variability of glial morphology, and expression profiles are all complications that can arise when designing an experiment using animal models. In general, many studies appear to focus on the acute phase of TBI, using mostly males to avoid variability in experimental groups, and mainly young adult animals, despite the high occurrence of TBI in the elderly and the higher possibility of worse outcomes post-TBI. We also noted a lack of articles with a focus on NG2-glia and oligodendrocyte functions in rmTBI.
13.2 Long-term consequences of traumatic brain injury
As aforementioned, many studies appear to focus on the acute phase of TBI. However, TBI should be handled more as a “chronic disease.” Various studies using data from both patients and animal models show long-term consequences of TBI, including physiological and/or cognitive impact. For example, a study from 2022 shows that up to 56% of patients with mTBI do not fully recover after 6 months (Madhok et al., 2022). Additionally, TBI is linked to several neurodegenerative diseases, such as AD, PD, or CTE, which was proved by population-based studies and confirmed by following animal experiments. Therefore, an effective and early treatment of TBI, based on understanding the chronic effects, could potentially shorten the recovery period, and limit lifelong consequences, as well as delay the onset of neurodegenerative diseases or alleviate their symptoms.
13.3 Traumatic brain injury diagnostics and treatment
For TBI patients, it is crucial to be properly diagnosed and treated as soon as possible. However, patients are still treated based on the severity of TBI, while neglecting the type of TBI and its pathologies. To distinguish them requires specific biomarkers, which would be specific to TBI, easy and fast to test, and become a stepping stone to accurate diagnosis (e.g., assist the severity or phase of TBI). Data available from biomarker testing would also help in research for more precise analysis. In 2023, U.S. Food and Drug Administration (FDA) approved the use of enzyme-linked immunosorbent assay testing GFAP and UCH-L1 presence in blood, to determine the need for CT or MRI scan.3 However, the need for improved diagnostic tools is still actual.
The existing commonly used therapy of TBI is causal but mostly unspecific (for example treatment of brain edema using mannitol, corticoids and diuretics) and does not aim directly at molecular and cellular mechanisms of the brain injury. It is therefore crucial to develop more sophisticated up-to-date approaches, similar to the glia-oriented search for therapeutical targets in treatment of stroke (Hernandez et al., 2021).
In TBI, glia-oriented senolytic therapies seem to be very promising not only in animal experiments but even in clinical trials. Senolytic and senomorphic therapies aim to improve cognitive outcomes by removing or modifying senescent cells. Efforts to identify senotherapeutic agents, have led to the discovery of various classes of senolytics, including kinase inhibitors, Bcl-2 family inhibitors, natural compounds, p53 inhibitors, heat shock protein 90 inhibitors, and histone deacetylase inhibitors (HDAC). Given the potential acceleration of aging after TBI, these approaches may offer innovative strategies to mitigate long-term deleterious effects. Dasatinib, an FDA-approved tyrosine kinase inhibitor, and quercetin, a natural flavonoid, induce apoptosis in senescent cells. Their combination removes senescent cells in vivo, improving outcomes in fibrotic pulmonary disease, age-associated bone loss, and physical function in old age (Schafer et al., 2017; Xu et al., 2018). Quercetin benefits in rodent TBI, reducing oxidative stress, enhancing mitochondrial function, and mitigating gliosis and anxious behavior (Yang et al., 2014; Li et al., 2016; Kosari-Nasab et al., 2019). Enzogenol, a quercetin-containing extract, demonstrated safety, tolerance, and potential cognitive benefits for up to a year post-mild TBI (Theadom et al., 2013; Walter et al., 2017). Curcumin, a turmeric compound, exhibits potent senolytic activity with anti-aging effects (Beltzig et al., 2021). Curcumin analogs, o-vanillin, and EF24 also display senolytic properties (Li et al., 2019). Curcumin administration reduces inflammation, glial activation, and improves cognitive outcomes in rodent TBI models (Sun et al., 2020; Khayatan et al., 2022). Additionally, curcumin protects against neurodegeneration in animal models (Ojha et al., 2012; Pluta et al., 2022) and shows benefits for critically ill TBI patients (Zahedi et al., 2021). Luteolin, another potential senolytic, improves cognitive function in moderate TBI patients (Campolo et al., 2021). Fisetin, a natural flavonol in foods like strawberries and apples, exhibits senolytic activity by targeting anti-apoptotic Bcl-2 family members (Zhu et al., 2017). Although not explored in clinical TBI, fisetin suppresses neuronal loss after experimental TBI and reduces neurodegeneration in experimental models (Zhang et al., 2018; Hassan et al., 2022). Experimental Bcl-2/Bcl-xL inhibitor Navitoclax (ABT263) improved cognitive function in male mice after rmTBI, indicating potential sex differences in neurodegeneration mechanisms (Schwab et al., 2022). The impact of Bcl-2 family inhibitors after moderate-severe TBI or in clinical populations remains unexplored. While promising, further research and clinical trials are necessary to establish senolytics’ mechanisms and efficacy in enhancing cognitive outcomes after TBI.
Microglial depletion and repopulation methods offer a promising technique for elucidating microglial biology (Barnett et al., 2021). The depletion of microglia has been investigated as an experimental therapeutic approach aimed at improving, preventing, or reversing neurocognitive deficits in various neuroinflammation-related disorders, including TBI (Dagher et al., 2015; Feng et al., 2016; Henry et al., 2020; Willis et al., 2020). The experimental removal of microglia from the CNS environment, achieved through pharmacological approaches such as administering colony-stimulating factor 1 receptor (CSF1R) inhibitors (Rice et al., 2015) or genetic induction of the diphtheria toxin receptor in microglia (Lund et al., 2018), represent a valuable tool for studying microglial contributions to TBI neuropathology. CSF1R inhibitors such as PLX5622 hinder the CSF1-dependent microglia trophic support. Consequently, administration of these inhibitors in the diet results in the depletion of over 90% of microglia in vivo within about 3 weeks (Rice et al., 2015). Preemptive microglial depletion, achieved through PLX5622 treatment or genetic manipulation prior to experimental TBI, has been shown to be beneficial in TBI-related spatial memory loss. This approach also stimulated functional neurogenesis in the hippocampus. However, continuous or delayed microglial depletion and repopulation failed to alleviate TBI-induced deficits, highlighting the crucial role of acutely timed or preemptive microglial treatment (Willis et al., 2020). In contrast, a separate study showed that PLX5622 administration 1 week after TBI reduced impairments in recognition and spatial memory, as well as neuropathological changes (Henry et al., 2020).
Another glia-oriented approach takes advantage of the stem cell properties of reactive astrocytes and NG2-glia, for their direct in vivo reprogramming functional neurons. Previous studies showed that some reactive astrocytes after injury may acquire stem cell-related properties, forming neurospheres in vitro (Lang et al., 2004; Buffo et al., 2008; Shimada et al., 2012; Guo et al., 2014). In contrast, reactive astrocytes generated glial cells (astrocytes and oligodendrocytes) but they typically did not produce neurons in vivo (Buffo et al., 2008; Shimada et al., 2012). Forced expression of NeuroD1 in reactive astrocytes helped overcome trans-lineage barriers. NeuroD1, crucial for adult neurogenesis, induces terminal neuronal differentiation (Boutin et al., 2010) and aids in reprogramming human fibroblasts into neurons (Pang et al., 2011). This theory was proven in vitro, when after the infection of human astrocytes by GFAP of:NeuroD1-IRES-GFP retrovirus, NeuroD1-positive cells also express positivity for NeuN, a marker of functional neurons (Guo et al., 2014). Another experiments of the same study showed that NeuroD1 may initiate a direct reprogramming of reactive glial cells post-brain injury into functional neurons in the mouse brain in vivo. The simultaneous generation of both excitatory and inhibitory neurons by NeuroD1 alone could potentially balance excitation and inhibition in the cortex after reprogramming (Guo et al., 2014).
Guo et al. (2014) demonstrated that the expression of NeuroD1 leads primarily to reprogramming of reactive astrocytes into glutamatergic neurons (Guo et al., 2014; Chen et al., 2020), while NeuroD1-positive NG2 cells can mature into both glutamatergic and GABAergic neurons (Guo et al., 2014). NG2 cells are major proliferative glial cells in the adult brain under normal conditions (Buffo et al., 2008; Kang et al., 2010). In the stab injury model, Guo et al. (2014) observed that the majority of cells with incorporation of NeuroD1 via CAG-GFP retrovirus were GFAP+, while NG2 cells created approximately 20% of the total infected cells (Guo et al., 2014). The authors suggested that different glial cells may be associated with different neuronal fate in lineage differentiation terms.
Other transcription factors, or their combinations, were also shown to induce conversion of astrocytes or NG2-glia into neurons both in vitro and in vivo: for example Neurog2 (Neurogenin2) (Heinrich et al., 2010; Grande et al., 2013), SOX2 (SRY-box transcription factor 2) (Niu et al., 2013; Heinrich et al., 2014), Ascl1 (Achaete-scute family BHLH transcription factor 1) (Liu et al., 2015; Torper et al., 2015), Brn2 (POU domain transcription factor Brn-2) (Torper et al., 2015), Myt1l (Myelin transcription factor 1 like) (Torper et al., 2015), or Dlx2 (Distal-less homeobox 2) (Heinrich et al., 2010). In contrast to the Guo et al. (2014), other studies showed that the lineage of glial cell is not crucial for determination of neuronal fate, and that different transcription factors may induce reprogramming of astrocytes or NG2-glia in both glutamatergic and GABAergic neurons (Heinrich et al., 2014; Torper et al., 2015).
The additional therapeutic targets are the SUR1 and TRPM4 channels, that play a crucial role in secondary injury processes post-TBI (see chapter Edema). The SUR1-TRPM4 complex, normally absent in the brain, becomes upregulated in various CNS cell types post-TBI (neurons, microglia, astrocytes, endothelial cells) (Simard et al., 2009; Gerzanich et al., 2019). Targeted inhibition of the SUR1-TRPM4 complex has demonstrated an efficacy in reducing edema and hemorrhage progression in preclinical contusional TBI models (Jha et al., 2020). Glibenclamide, also known as glyburide, acts as an antagonist for SUR1-TRPM4 and is already widely used in treating type 2 diabetes (Jha et al., 2021). Glibenclamide inhibits calcium influx, preventing depolarization and swelling in neurons, glial cells, and vascular endothelial cells following brain injury (King et al., 2018; Jha et al., 2020). Glibenclamide has proven positive effects during BBB breakdown or edema following cerebral hemorrhage, and reduces mortality in TBI, however, its impact on microglial activation and inflammatory cell infiltration remains unclear (Simard et al., 2009; Ortega et al., 2013; DeWitt et al., 2018; Shiokawa et al., 2022). While the initial research focused on the glibenclamide effect in ischemic stroke, mounting evidence underscores the central role of SUR1-TRPM4 also in secondary TBI injuries, particularly in contusional edema and hemorrhage progression (Jha et al., 2020, 2021). Glibenclamide, noted for its pleiotropic protective effects in TBI animal models, is currently the subject of a phase II clinical trial (NCT01454154). Further details can be found at https://clinicaltrials.gov/ct2/show/NCT01454154 (Lerouet et al., 2021).
Particularly noteworthy for the modern treatment of TBI can be the use of cell therapy, employing mesenchymal stromal cells (MSCs) or amnion-derived multipotent progenitor cells (AMPs) for enhancement of neurological recovery. The effect of administration of AMPs has been evaluated in PBBI model (Chen et al., 2009). The treatment significantly attenuated axonal degeneration in both the thalamus and the corpus callosum even though no significant difference in an injury volume was observed across all treatment groups. None of the labeled AMP cells appeared to express neural differentiation, as evidenced by the lack of double labeling with nestin, S-100, GFAP, and microtubule-associated protein 2 (MAP-2) immunostaining. AMP cell migration was specifically induced by PBBI and required subventricular zone homing, yet the neuroprotective effect of intracerebral ventrical treatment using AMP cells was not limited to the area where the cells were present (Chen et al., 2009). The ability of MSCs to promote neurite outgrowth and intrinsic neurogenesis after TBI was demonstrated in both animal models and early phase clinical studies (Zhang et al., 2015). Originally, it was thought that the recovery from brain injury following MSC therapy resulted from the replacement of damaged tissue by differentiated MSCs. However, subsequent studies offer evidence that the therapeutic effectiveness of MSCs in treating TBI is predominantly linked to the vigorous generation and release of EVs, notably exosomes (Zhang et al., 2015, 2017a,2017b; Xiong et al., 2017). Interaction of exosomes containing miRNA with brain parenchymal cells and the neurogenic niche promotes neurogenesis and brain remodeling, suggesting a potential benefit in the treatment of TBI. Exosomes also emerge as potent candidate biomarkers for mTBI (Atif and Hicks, 2019; Pinchi et al., 2020). Additionally, miRNAs have been identified as therapeutic targets for neurorehabilitation with reported efficacy in reducing brain edema and inflammation (Sun et al., 2018).
Overall, the study of TBI and its understanding are moving forward, with new models and techniques being developed. The future seek of new therapeutical targets should involve research of factors and signaling pathways affecting mechanisms of neuroinflammation, in both acute and chronic phases, as well as research focusing on the RNA field, specifically miRNA, which shows substantial results in other areas, including cancer studies. TBI treatment could be personalized in the future, considering the patient’s overall health, age, or sex. Additionally, a more accurate estimation of TBI, as well as a unified and precise classification (e.g., glial cell phenotype or TBI severity assessment) combined with gene analysis of individual subpopulations found in individual types of TBI, would overall contribute to the simplification of data processing and research progress acceleration.
14 Conclusion
Traumatic brain injury is one of the most common brain injuries with a high occurrence of mortality every year, and those who survive TBI suffer from long-term unfavorable consequences. The relevance of glial cells in TBI research has been well-known for a long time and is established by numerous published articles. This systematic review provides a comprehensive overview of the existing literature on reactive gliosis in TBI. Through a thorough analysis of numerous studies, we have synthesized the current knowledge regarding the complex cellular and molecular processes underlying reactive gliosis following TBI. Our findings highlight the multifaceted nature of reactive gliosis, showcasing its dual roles as both a neuroprotective and potentially detrimental response to TBI. While reactive gliosis may contribute to tissue repair, immune modulation, and the restoration of homeostasis, it can also lead to secondary neuroinflammation and exacerbate neurological deficits.
A deeper understanding of reactive gliosis is essential for the development of targeted therapies that can mitigate its negative effects while harnessing its neuroprotective potential. By continuing to unravel the complexities of reactive gliosis in TBI, we can strive to improve the prognosis and quality of life for individuals affected by this devastating condition. This review summarizes the key points and highlights the importance of further research in this area.
Author contributions
ZA: Conceptualization, Funding acquisition, Visualization, Writing – original draft. MC: Conceptualization, Visualization, Writing – original draft. MA: Funding acquisition, Resources, Validation, Writing – review and editing. LV: Conceptualization, Supervision, Validation, Visualization, Writing – review and editing.
Funding
The author(s) declare financial support was received for the research, authorship, and/or publication of this article. This study was supported by grant 23-06269S (MA) from the Czech Science Foundation and 238023 (ZA) from the Charles University Grant Agency.
Acknowledgments
We would like to thank Frances Zatrepalkova for proofreading the manuscript.
Conflict of interest
The authors declare that the research was conducted in the absence of any commercial or financial relationships that could be construed as a potential conflict of interest.
Publisher’s note
All claims expressed in this article are solely those of the authors and do not necessarily represent those of their affiliated organizations, or those of the publisher, the editors and the reviewers. Any product that may be evaluated in this article, or claim that may be made by its manufacturer, is not guaranteed or endorsed by the publisher.
Supplementary material
The Supplementary Material for this article can be found online at: https://www.frontiersin.org/articles/10.3389/fncel.2024.1335849/full#supplementary-material
Footnotes
- ^ https://www.who.int/news-room/fact-sheets/detail/dementia
- ^ https://health.mil/Military-Health-Topics/Centers-of-Excellence/Traumatic-Brain-Injury-Center-of-Excellence/DOD-TBI-Worldwide-Numbers
- ^ https://www.accessdata.fda.gov/scripts/cdrh/cfdocs/cfpmn/pmn.cfm?ID=K223602
References
Aalinkeel, R., and Mahajan, S. D. (2016). Neuroprotective role of galectin-1 in central nervous system pathophysiology. Neural Regen. Res. 11, 896–897. doi: 10.4103/1673-5374.184455
Abdul-Muneer, P. M., Long, M., Conte, A. A., Santhakumar, V., and Pfister, B. J. (2017). High Ca(2 +) influx during traumatic brain injury leads to caspase-1-dependent neuroinflammation and cell death. Mol Neurobiol. 54, 3964–3975. doi: 10.1007/s12035-016-9949-4
Abe, N., Choudhury, M. E., Watanabe, M., Kawasaki, S., Nishihara, T., Yano, H., et al. (2018). Comparison of the detrimental features of microglia and infiltrated macrophages in traumatic brain injury: A study using a hypnotic bromovalerylurea. Glia 66, 2158–2173. doi: 10.1002/glia.23469
Acaz-Fonseca, E., Duran, J. C., Carrero, P., Garcia-Segura, L. M., and Arevalo, M. A. (2015). Sex differences in glia reactivity after cortical brain injury. Glia 63, 1966–1981. doi: 10.1002/glia.22867
Adams, K. L., and Gallo, V. (2018). The diversity and disparity of the glial scar. Nat. Neurosci. 21, 9–15. doi: 10.1038/s41593-017-0033-9
Ahmed, F., Gyorgy, A., Kamnaksh, A., Ling, G., Tong, L., Parks, S., et al. (2012). Time-dependent changes of protein biomarker levels in the cerebrospinal fluid after blast traumatic brain injury. Electrophoresis 33, 3705–3711. doi: 10.1002/elps.201200299
Ahmed, M. E., Selvakumar, G. P., Kempuraj, D., Raikwar, S. P., Thangavel, R., Bazley, K., et al. (2020). Glia Maturation Factor (GMF) regulates microglial expression phenotypes and the associated neurological deficits in a mouse model of traumatic brain injury. Mol. Neurobiol. 57, 4438–4450. doi: 10.1007/s12035-020-02040-y
Akay, L. A., Effenberger, A. H., and Tsai, L. H. (2021). Cell of all trades: oligodendrocyte precursor cells in synaptic, vascular, and immune function. Genes Dev. 35, 180–198. doi: 10.1101/gad.344218.120
Alibhai, J. D., Diack, A. B., and Manson, J. C. (2018). Unravelling the glial response in the pathogenesis of Alzheimer’s disease. FASEB J. 32, 5766–5777. doi: 10.1096/fj.201801360R
Allen, G. V., Gerami, D., and Esser, M. J. (2000). Conditioning effects of repetitive mild neurotrauma on motor function in an animal model of focal brain injury. Neuroscience 99, 93–105. doi: 10.1016/s0306-4522(00)00185-8
Amiry-Moghaddam, M., Frydenlund, D. S., and Ottersen, O. P. (2004). Anchoring of aquaporin-4 in brain: Molecular mechanisms and implications for the physiology and pathophysiology of water transport. Neuroscience 129, 999–1010. doi: 10.1016/j.neuroscience.2004.08.049
Amorini, A. M., Lazzarino, G., Di Pietro, V., Signoretti, S., Lazzarino, G., Belli, A., et al. (2017). Severity of experimental traumatic brain injury modulates changes in concentrations of cerebral free amino acids. J. Cell Mol. Med. 21, 530–542. doi: 10.1111/jcmm.12998
Anderson, M. A., Burda, J. E., Ren, Y., Ao, Y., O’Shea, T. M., Kawaguchi, R., et al. (2016). Astrocyte scar formation aids central nervous system axon regeneration. Nature 532, 195–200. doi: 10.1038/nature17623
Androvic, P., Kirdajova, D., Tureckova, J., Zucha, D., Rohlova, E., Abaffy, P., et al. (2020). Decoding the transcriptional response to ischemic stroke in young and aged mouse brain. Cell Rep. 31:107777. doi: 10.1016/j.celrep.2020.107777
Angeloni, C., Prata, C., Dalla Sega, F. V., Piperno, R., and Hrelia, S. (2015). Traumatic brain injury and NADPH oxidase: a deep relationship. Oxid. Med. Cell Longev. 2015:370312. doi: 10.1155/2015/370312
Antunes, A., and Martins-de-Souza, D. (2023). Single-Cell RNA sequencing and its applications in the study of psychiatric disorders. Biol. Psychiatry Glob. Open Sci. 3, 329–339. doi: 10.1016/j.bpsgos.2022.03.013
Araki, T., Yokota, H., and Morita, A. (2017). Pediatric traumatic brain injury: characteristic features, diagnosis, and management. Neurol. Med. Chir. 57, 82–93. doi: 10.2176/nmc.ra.2016-0191
Arevalo, M. A., Santos-Galindo, M., Bellini, M. J., Azcoitia, I., and Garcia-Segura, L. M. (2010). Actions of estrogens on glial cells: Implications for neuroprotection. Biochim. Biophys. Acta 1800, 1106–1112. doi: 10.1016/j.bbagen.2009.10.002
Armand, E. J., Li, J., Xie, F., Luo, C., and Mukamel, E. A. (2021). Single-cell sequencing of brain cell transcriptomes and epigenomes. Neuron 109, 11–26. doi: 10.1016/j.neuron.2020.12.010
Arneson, D., Zhang, G., Ahn, I. S., Ying, Z., Diamante, G., Cely, I., et al. (2022). Systems spatiotemporal dynamics of traumatic brain injury at single-cell resolution reveals humanin as a therapeutic target. Cell Mol. Life Sci. 79:480. doi: 10.1007/s00018-022-04495-9
Arneson, D., Zhang, G., Ying, Z., Zhuang, Y., Byun, H. R., Ahn, I. S., et al. (2018). Single cell molecular alterations reveal target cells and pathways of concussive brain injury. Nat. Commun. 9:3894. doi: 10.1038/s41467-018-06222-0
Arun, P., Rossetti, F., Wilder, D. M., Sajja, S., Van Albert, S. A., Wang, Y., et al. (2020). Blast exposure leads to accelerated cellular senescence in the rat brain. Front. Neurol. 11:438. doi: 10.3389/fneur.2020.00438
Asadi-Pooya, A. A., and Farazdaghi, M. (2021). Is severe head injury associated with functional (psychogenic) seizures? Seizure 89, 38–40. doi: 10.1016/j.seizure.2021.04.018
Asher, R. A., Morgenstern, D. A., Moon, L. D., and Fawcett, J. W. (2001). Chondroitin sulphate proteoglycans: inhibitory components of the glial scar. Prog. Brain Res. 132, 611–619. doi: 10.1016/S0079-6123(01)32106-4
Asher, R. A., Morgenstern, D. A., Shearer, M. C., Adcock, K. H., Pesheva, P., and Fawcett, J. W. (2002). Versican is upregulated in CNS injury and is a product of oligodendrocyte lineage cells. J. Neurosci. 22, 2225–2236. doi: 10.1523/jneurosci.22-06-02225.2002
Atif, H., and Hicks, S. D. (2019). A Review of MicroRNA Biomarkers in Traumatic Brain Injury. J. Exp. Neurosci. 13:1179069519832286. doi: 10.1177/1179069519832286
Aungst, S. L., Kabadi, S. V., Thompson, S. M., Stoica, B. A., and Faden, A. I. (2014). Repeated mild traumatic brain injury causes chronic neuroinflammation, changes in hippocampal synaptic plasticity, and associated cognitive deficits. J. Cereb. Blood Flow Metab. 34, 1223–1232. doi: 10.1038/jcbfm.2014.75
Bachstetter, A. D., Rowe, R. K., Kaneko, M., Goulding, D., Lifshitz, J., and Van Eldik, L. J. (2013). The p38alpha MAPK regulates microglial responsiveness to diffuse traumatic brain injury. J. Neurosci. 33, 6143–6153. doi: 10.1523/JNEUROSCI.5399-12.2013
Bachstetter, A. D., Xing, B., de Almeida, L., Dimayuga, E. R., Watterson, D. M., and Van Eldik, L. J. (2011). Microglial p38alpha MAPK is a key regulator of proinflammatory cytokine up-regulation induced by toll-like receptor (TLR) ligands or beta-amyloid (Abeta). J. Neuroinflammation 8:79. doi: 10.1186/1742-2094-8-79
Baker, D. J., and Petersen, R. C. (2018). Cellular senescence in brain aging and neurodegenerative diseases: evidence and perspectives. J. Clin. Invest. 128, 1208–1216. doi: 10.1172/JCI95145
Barnes, C. A. (1979). Memory deficits associated with senescence: a neurophysiological and behavioral study in the rat. J. Comp. Physiol. Psychol. 93, 74–104. doi: 10.1037/h0077579
Barnett, A. M., Crews, F. T., and Coleman, L. G. (2021). Microglial depletion and repopulation: a new era of regenerative medicine? Neural Regen. Res. 16, 1204–1205. doi: 10.4103/1673-5374.300439
Bashir, A., Abebe, Z. A., McInnes, K. A., Button, E. B., Tatarnikov, I., Cheng, W. H., et al. (2020). Increased severity of the CHIMERA model induces acute vascular injury, sub-acute deficits in memory recall, and chronic white matter gliosis. Exp. Neurol. 324:113116. doi: 10.1016/j.expneurol.2019.113116
Basurco, L., Abellanas, M. A., Ayerra, L., Conde, E., Vinueza-Gavilanes, R., Luquin, E., et al. (2023). Microglia and astrocyte activation is region-dependent in the alpha-synuclein mouse model of Parkinson’s disease. Glia 71, 571–587. doi: 10.1002/glia.24295
Bellezza, I., Giambanco, I., Minelli, A., and Donato, R. (2018). Nrf2-Keap1 signaling in oxidative and reductive stress. Biochim. Biophys. Acta Mol. Cell Res. 1865, 721–733. doi: 10.1016/j.bbamcr.2018.02.010
Bellver-Landete, V., Bretheau, F., Mailhot, B., Vallieres, N., Lessard, M., Janelle, M. E., et al. (2019). Microglia are an essential component of the neuroprotective scar that forms after spinal cord injury. Nat. Commun. 10:518. doi: 10.1038/s41467-019-08446-0
Benfenati, V., Caprini, M., Dovizio, M., Mylonakou, M. N., Ferroni, S., Ottersen, O. P., et al. (2011). An aquaporin-4/transient receptor potential vanilloid 4 (AQP4/TRPV4) complex is essential for cell-volume control in astrocytes. Proc. Natl. Acad. Sci. U. S. A. 108, 2563–2568. doi: 10.1073/pnas.1012867108
Bennett, I. J., Madden, D. J., Vaidya, C. J., Howard, D. V., and Howard, J. H. Jr. (2010). Age-related differences in multiple measures of white matter integrity: A diffusion tensor imaging study of healthy aging. Hum. Brain Mapp. 31, 378–390. doi: 10.1002/hbm.20872
Bennett, R. E., Mac Donald, C. L., and Brody, D. L. (2012). Diffusion tensor imaging detects axonal injury in a mouse model of repetitive closed-skull traumatic brain injury. Neurosci. Lett. 513, 160–165. doi: 10.1016/j.neulet.2012.02.024
Beschorner, R., Dietz, K., Schauer, N., Mittelbronn, M., Schluesener, H. J., Trautmann, K., et al. (2007). Expression of EAAT1 reflects a possible neuroprotective function of reactive astrocytes and activated microglia following human traumatic brain injury. Histol. Histopathol. 22, 515–526. doi: 10.14670/HH-22.515
Beltzig, L., Frumkina, A., Schwarzenbach, C., and Kaina, B. (2021). Cytotoxic, Genotoxic and Senolytic Potential of Native and Micellar Curcumin. Nutrients 13. doi: 10.3390/nu13072385
Bhalala, O. G., Pan, L., Sahni, V., McGuire, T. L., Gruner, K., Tourtellotte, W. G., et al. (2012). microRNA-21 regulates astrocytic response following spinal cord injury. J. Neurosci. 32, 17935–17947. doi: 10.1523/JNEUROSCI.3860-12.2012
Biegon, A. (2021). Considering Biological Sex in Traumatic Brain Injury. Front. Neurol. 12:576366. doi: 10.3389/fneur.2021.576366
Bodnar, C. N., Roberts, K. N., Higgins, E. K., and Bachstetter, A. D. (2019). A systematic review of closed head injury models of mild traumatic brain injury in mice and rats. J. Neurotrauma 36, 1683–1706. doi: 10.1089/neu.2018.6127
Bolton, A. N., and Saatman, K. E. (2014). Regional neurodegeneration and gliosis are amplified by mild traumatic brain injury repeated at 24-hour intervals. J. Neuropathol. Exp. Neurol. 73, 933–947. doi: 10.1097/NEN.0000000000000115
Bonifacino, T., Zerbo, R. A., Balbi, M., Torazza, C., Frumento, G., Fedele, E., et al. (2021). Nearly 30 years of animal models to study amyotrophic lateral sclerosis: A historical overview and future perspectives. Int. J. Mol. Sci. 22:12236. doi: 10.3390/ijms222212236
Bonnier, C., Mesples, B., and Gressens, P. (2004). Animal models of shaken baby syndrome: revisiting the pathophysiology of this devastating injury. Pediatr. Rehabil. 7, 165–171. doi: 10.1080/13638490410001703325
Borst, K., Dumas, A. A., and Prinz, M. (2021). Microglia: Immune and non-immune functions. Immunity 54, 2194–2208. doi: 10.1016/j.immuni.2021.09.014
Boutin, C., Hardt, O., de Chevigny, A., Core, N., Goebbels, S., Seidenfaden, R., et al. (2010). NeuroD1 induces terminal neuronal differentiation in olfactory neurogenesis. Proc. Natl. Acad. Sci. U. S. A. 107, 1201–1206. doi: 10.1073/pnas.0909015107
Boza-Serrano, A., Reyes, J. F., Rey, N. L., Leffler, H., Bousset, L., Nilsson, U., et al. (2014). The role of Galectin-3 in alpha-synuclein-induced microglial activation. Acta Neuropathol. Commun. 2:156. doi: 10.1186/s40478-014-0156-0
Brambrink, A. M., Back, S. A., Riddle, A., Gong, X., Moravec, M. D., Dissen, G. A., et al. (2012). Isoflurane-induced apoptosis of oligodendrocytes in the neonatal primate brain. Ann. Neurol. 72, 525–535. doi: 10.1002/ana.23652
Bramlett, H. M., and Dietrich, W. D. (2001). Neuropathological protection after traumatic brain injury in intact female rats versus males or ovariectomized females. J. Neurotrauma 18, 891–900. doi: 10.1089/089771501750451811
Brazinova, A., Rehorcikova, V., Taylor, M. S., Buckova, V., Majdan, M., Psota, M., et al. (2021). Epidemiology of traumatic brain injury in europe: A living systematic review. J. Neurotrauma 38, 1411–1440. doi: 10.1089/neu.2015.4126
Brett, B. L., Gardner, R. C., Godbout, J., Dams-O’Connor, K., and Keene, C. D. (2022). Traumatic Brain Injury and Risk of Neurodegenerative Disorder. Biol. Psychiatry 91, 498–507. doi: 10.1016/j.biopsych.2021.05.025
Briggs, D. I., Angoa-Perez, M., and Kuhn, D. M. (2016). Prolonged Repetitive Head Trauma Induces a Singular Chronic Traumatic Encephalopathy-Like Pathology in White Matter Despite Transient Behavioral Abnormalities. Am. J. Pathol. 186, 2869–2886. doi: 10.1016/j.ajpath.2016.07.013
Browne, K. D., Chen, X. H., Meaney, D. F., and Smith, D. H. (2011). Mild traumatic brain injury and diffuse axonal injury in swine. J. Neurotrauma 28, 1747–1755. doi: 10.1089/neu.2011.1913
Bryden, D. W., Tilghman, J. I., and Hinds, S. R. II (2019). Blast-Related Traumatic Brain Injury: Current Concepts and Research Considerations. J. Exp. Neurosci. 13:1179069519872213. doi: 10.1177/1179069519872213
Bugay, V., Bozdemir, E., Vigil, F. A., Chun, S. H., Holstein, D. M., Elliott, W. R., et al. (2020). A mouse model of repetitive blast traumatic brain injury reveals post-trauma seizures and increased neuronal excitability. J. Neurotrauma 37, 248–261. doi: 10.1089/neu.2018.6333
Buffo, A., Rite, I., Tripathi, P., Lepier, A., Colak, D., Horn, A. P., et al. (2008). Origin and progeny of reactive gliosis: A source of multipotent cells in the injured brain. Proc. Natl. Acad. Sci. U. S. A. 105, 3581–3586. doi: 10.1073/pnas.0709002105
Burda, J. E., and Sofroniew, M. V. (2014). Reactive gliosis and the multicellular response to CNS damage and disease. Neuron 81, 229–248. doi: 10.1016/j.neuron.2013.12.034
Burguillos, M. A., Svensson, M., Schulte, T., Boza-Serrano, A., Garcia-Quintanilla, A., Kavanagh, E., et al. (2015). Microglia-Secreted Galectin-3 Acts as a Toll-like Receptor 4 Ligand and Contributes to Microglial Activation. Cell Rep. 10, 1626–1638. doi: 10.1016/j.celrep.2015.02.012
Butt, A. M., De La Rocha, I. C., and Rivera, A. (2019). Oligodendroglial Cells in Alzheimer’s Disease. Adv. Exp. Med. Biol. 1175, 325–333. doi: 10.1007/978-981-13-9913-8_12
Cai, W., Yang, T., Liu, H., Han, L., Zhang, K., Hu, X., et al. (2018). Peroxisome proliferator-activated receptor gamma (PPARgamma): A master gatekeeper in CNS injury and repair. Prog. Neurobiol. 16, 27–58. doi: 10.1016/j.pneurobio.2017.10.002
Calabresi, P., Mechelli, A., Natale, G., Volpicelli-Daley, L., Di Lazzaro, G., and Ghiglieri, V. (2023). Alpha-synuclein in Parkinson’s disease and other synucleinopathies: from overt neurodegeneration back to early synaptic dysfunction. Cell Death Dis. 14:176. doi: 10.1038/s41419-023-05672-9
Calafatti, M., Cocozza, G., Limatola, C., and Garofalo, S. (2023). Microglial crosstalk with astrocytes and immune cells in amyotrophic lateral sclerosis. Front. Immunol. 14:1223096. doi: 10.3389/fimmu.2023.1223096
Camacho-Soto, A., Warden, M. N., Searles Nielsen, S., Salter, A., Brody, D. L., Prather, H., et al. (2017). Traumatic brain injury in the prodromal period of Parkinson’s disease: A large epidemiological study using medicare data. Ann. Neurol. 82, 744–754. doi: 10.1002/ana.25074
Campolo, M., Crupi, R., Cordaro, M., Cardali, S. M., Ardizzone, A., Casili, G., et al. (2021). Co-Ultra PEALut enhances endogenous repair response following moderate traumatic brain injury. Int. J. Mol. Sci. 22:8717. doi: 10.3390/ijms22168717
Campos-Pires, R., Koziakova, M., Yonis, A., Pau, A., Macdonald, W., Harris, K., et al. (2018). Xenon protects against blast-induced traumatic brain injury in an in vitro model. J. Neurotrauma 35, 1037–1044. doi: 10.1089/neu.2017.5360
Campos-Pires, R., Ong, B. E., Koziakova, M., Ujvari, E., Fuller, I., Boyles, C., et al. (2023). Repetitive, but not single, mild blast TBI causes persistent neurological impairments and selective cortical neuronal loss in rats. Brain Sci. 13:1298. doi: 10.3390/brainsci13091298
Capizzi, A., Woo, J., and Verduzco-Gutierrez, M. (2020). Traumatic brain injury: An overview of epidemiology, pathophysiology, and medical management. Med. Clin. North Am. 104, 213–238. doi: 10.1016/j.mcna.2019.11.001
Caplan, H. W., Cardenas, F., Gudenkauf, F., Zelnick, P., Xue, H., Cox, C. S., et al. (2020). Spatiotemporal distribution of microglia after traumatic brain injury in male mice. ASN Neuro. 12:1759091420911770. doi: 10.1177/1759091420911770
Cargnello, M., and Roux, P. P. (2011). Activation and function of the MAPKs and their substrates, the MAPK-activated protein kinases. Microbiol. Mol. Biol. Rev. 75, 50–83. doi: 10.1128/MMBR.00031-10
Castejon, O. J. (1998). Morphological astrocytic changes in complicated human brain trauma. A light and electron microscopic study. Brain Inj. 12, 409–427. doi: 10.1080/026990598122539
Castro, L., Noelia, M., Vidal-Jorge, M., Sanchez-Ortiz, D., Gandara, D., Martinez-Saez, E., et al. (2019). Kir6.2, the pore-forming subunit of ATP-Sensitive K(+) channels, is overexpressed in human posttraumatic brain contusions. J. Neurotrauma 36, 165–175. doi: 10.1089/neu.2017.5619
Chamoun, R., Suki, D., Gopinath, S. P., Goodman, J. C., and Robertson, C. (2010). Role of extracellular glutamate measured by cerebral microdialysis in severe traumatic brain injury. J. Neurosurg. 113, 564–570. doi: 10.3171/2009.12.JNS09689
Chanti-Ketterl, M., Pieper, C. F., Yaffe, K., and Plassman, B. L. (2023). Associations between traumatic brain injury and cognitive decline among older male veterans: A twin study. Neurology 101, e1761–e1770. doi: 10.1212/WNL.0000000000207819
Chapman, J., Fielder, E., and Passos, J. F. (2019). Mitochondrial dysfunction and cell senescence: deciphering a complex relationship. FEBS Lett. 593, 1566–1579. doi: 10.1002/1873-3468.13498
Chen, M., Dong, Y., and Simard, J. M. (2003). Functional coupling between sulfonylurea receptor type 1 and a non-selective cation channel in reactive astrocytes from adult rat brain. J. Neurosci. 23, 8568–8577. doi: 10.1523/JNEUROSCI.23-24-08568.2003
Chen, M., and Simard, J. M. (2001). Cell swelling and a non-selective cation channel regulated by internal Ca2 + and ATP in native reactive astrocytes from adult rat brain. J. Neurosci. 21, 6512–6521. doi: 10.1523/JNEUROSCI.21-17-06512.2001
Chen, Y. C., Ma, N. X., Pei, Z. F., Wu, Z., Do-Monte, F. H., Keefe, S., et al. (2020). A NeuroD1 AAV-based gene therapy for functional brain repair after ischemic injury through in vivo astrocyte-to-neuron conversion. Mol. Ther. 28, 217–234. doi: 10.1016/j.ymthe.2019.09.003
Chen, Z., Tortella, F. C., Dave, J. R., Marshall, V. S., Clarke, D. L., Sing, G., et al. (2009). Human amnion-derived multipotent progenitor cell treatment alleviates traumatic brain injury-induced axonal degeneration. J. Neurotrauma 26, 1987–1997. doi: 10.1089/neu.2008.0863
Chen, Z. J., Negra, M., Levine, A., Ughrin, Y., and Levine, J. M. (2002). Oligodendrocyte precursor cells: reactive cells that inhibit axon growth and regeneration. J. Neurocytol. 31, 481–495. doi: 10.1023/a:1025791614468
Cherian, L., Hlatky, R., and Robertson, C. S. (2004). Nitric oxide in traumatic brain injury. Brain Pathol. 14, 195–201. doi: 10.1111/j.1750-3639.2004.tb00053.x
Cherry, J. D., Tripodis, Y., Alvarez, V. E., Huber, B., Kiernan, P. T., Daneshvar, D. H., et al. (2016). Microglial neuroinflammation contributes to tau accumulation in chronic traumatic encephalopathy. Acta Neuropathol. Commun. 4:112. doi: 10.1186/s40478-016-0382-8
Chini, C., Hogan, K. A., Warner, G. M., Tarrago, M. G., Peclat, T. R., Tchkonia, T., et al. (2019). The NADase CD38 is induced by factors secreted from senescent cells providing a potential link between senescence and age-related cellular NAD(+) decline. Biochem Biophys. Res. Commun. 513, 486–493. doi: 10.1016/j.bbrc.2019.03.199
Chmelova, M., Sucha, P., Bochin, M., Vorisek, I., Pivonkova, H., Hermanova, Z., et al. (2019). The role of aquaporin-4 and transient receptor potential vaniloid isoform 4 channels in the development of cytotoxic edema and associated extracellular diffusion parameter changes. Eur. J. Neurosci. 50, 1685–1699. doi: 10.1111/ejn.14338
Chopra, S., Shaw, M., Shaw, T., Sachdev, P. S., Anstey, K. J., and Cherbuin, N. (2018). More highly myelinated white matter tracts are associated with faster processing speed in healthy adults. Neuroimage 171, 332–340. doi: 10.1016/j.neuroimage.2017.12.069
Clark, R. S., Chen, J., Watkins, S. C., Kochanek, P. M., Chen, M., Stetler, R. A., et al. (1997). Apoptosis-suppressor gene bcl-2 expression after traumatic brain injury in rats. J. Neurosci. 17, 9172–9182. doi: 10.1523/JNEUROSCI.17-23-09172.1997
Clark, R. S., Kochanek, P. M., Watkins, S. C., Chen, M., Dixon, C. E., Seidberg, N. A., et al. (2000). Caspase-3 mediated neuronal death after traumatic brain injury in rats. J. Neurochem. 74, 740–753. doi: 10.1046/j.1471-4159.2000.740740.x
Clarke, L. E., Liddelow, S. A., Chakraborty, C., Munch, A. E., Heiman, M., and Barres, B. A. (2018). Normal aging induces A1-like astrocyte reactivity. Proc. Natl. Acad. Sci. U. S. A. 115, E1896–E1905. doi: 10.1073/pnas.1800165115
Clausen, F., Hanell, A., Israelsson, C., Hedin, J., Ebendal, T., Mir, A. K., et al. (2011). Neutralization of interleukin-1beta reduces cerebral edema and tissue loss and improves late cognitive outcome following traumatic brain injury in mice. Eur. J. Neurosci. 34, 110–123. doi: 10.1111/j.1460-9568.2011.07723.x
Cohen, J., and Torres, C. (2019). Astrocyte senescence: Evidence and significance. Aging Cell 18, e12937. doi: 10.1111/acel.12937
Conti, A. C., Raghupathi, R., Trojanowski, J. Q., and McIntosh, T. K. (1998). Experimental brain injury induces regionally distinct apoptosis during the acute and delayed post-traumatic period. J. Neurosci. 18, 5663–5672. doi: 10.1523/JNEUROSCI.18-15-05663.1998
Cornelius, C., Crupi, R., Calabrese, V., Graziano, A., Milone, P., Pennisi, G., et al. (2013). Traumatic brain injury: oxidative stress and neuroprotection. Antioxid. Redox Signal 19, 836–853. doi: 10.1089/ars.2012.4981
Corrigan, F., Wee, I. C., and Collins-Praino, L. E. (2023). Chronic motor performance following different traumatic brain injury severity-A systematic review. Front. Neurol. 14:1180353. doi: 10.3389/fneur.2023.1180353
Cuenda, A., and Rousseau, S. (2007). p38 MAP-kinases pathway regulation, function and role in human diseases. Biochim. Biophys. Acta 1773, 1358–1375. doi: 10.1016/j.bbamcr.2007.03.010
Culley, D. J., Cotran, E. K., Karlsson, E., Palanisamy, A., Boyd, J. D., Xie, Z., et al. (2013). Isoflurane affects the cytoskeleton but not survival, proliferation, or synaptogenic properties of rat astrocytes in vitro. Br. J. Anaesth. 110 Suppl, i19–i28. doi: 10.1093/bja/aet169
Dagher, N. N., Najafi, A. R., Kayala, K. M., Elmore, M. R., White, T. E., Medeiros, R., et al. (2015). Colony-stimulating factor 1 receptor inhibition prevents microglial plaque association and improves cognition in 3xTg-AD mice. J. Neuroinflammation 12:139. doi: 10.1186/s12974-015-0366-9
Davalos, D., Grutzendler, J., Yang, G., Kim, J. V., Zuo, Y., Jung, S., et al. (2005). ATP mediates rapid microglial response to local brain injury in vivo. Nat. Neurosci. 8, 752–758. doi: 10.1038/nn1472
de Boer, E. M. J., Orie, V. K., Williams, T., Baker, M. R., De Oliveira, H. M., Polvikoski, T., et al. (2020). TDP-43 proteinopathies: a new wave of neurodegenerative diseases. J. Neurol. Neurosurg. Psychiatry 92, 86–95. doi: 10.1136/jnnp-2020-322983
Delic, V., Beck, K. D., Pang, K. C. H., and Citron, B. A. (2020). Biological links between traumatic brain injury and Parkinson’s disease. Acta Neuropathol. Commun. 8:45. doi: 10.1186/s40478-020-00924-7
Deng, Y., Jiang, X., Deng, X., Chen, H., Xu, J., Zhang, Z., et al. (2020). Pioglitazone ameliorates neuronal damage after traumatic brain injury via the PPARgamma/NF-kappaB/IL-6 signaling pathway. Genes Dis. 7, 253–265. doi: 10.1016/j.gendis.2019.05.002
Dent, K. A., Christie, K. J., Bye, N., Basrai, H. S., Turbic, A., Habgood, M., et al. (2015). Oligodendrocyte birth and death following traumatic brain injury in adult mice. PLoS One 10:e0121541. doi: 10.1371/journal.pone.0121541
Deshetty, U. M., and Periyasamy, P. (2023). Potential biomarkers in experimental animal models for traumatic brain injury. J. Clin. Med. 12:3923. doi: 10.3390/jcm12123923
Dewan, M. C., Rattani, A., Gupta, S., Baticulon, R. E., Hung, Y. C., Punchak, M., et al. (2018). Estimating the global incidence of traumatic brain injury. J. Neurosurg 130, 1080–1097. doi: 10.3171/2017.10.JNS17352
DeWitt, D. S., Hawkins, B. E., Dixon, C. E., Kochanek, P. M., Armstead, W., Bass, C. R., et al. (2018). Pre-clinical testing of therapies for traumatic brain injury. J. Neurotrauma 35, 2737–2754. doi: 10.1089/neu.2018.5778
Dickerson, M. R., Bailey, Z. S., Murphy, S. F., Urban, M. J., and VandeVord, P. J. (2020). Glial activation in the thalamus contributes to vestibulomotor deficits following blast-induced neurotrauma. Front. Neurol. 11:618. doi: 10.3389/fneur.2020.00618
Dimou, L., and Gallo, V. (2015). NG2-glia and their functions in the central nervous system. Glia 63, 1429–1451. doi: 10.1002/glia.22859
Dixon, C. E., Kochanek, P. M., Yan, H. Q., Schiding, J. K., Griffith, R. G., Baum, E., et al. (1999). One-year study of spatial memory performance, brain morphology, and cholinergic markers after moderate controlled cortical impact in rats. J. Neurotrauma 16, 109–122. doi: 10.1089/neu.1999.16.109
Dixon, C. E., Lyeth, B. G., Povlishock, J. T., Findling, R. L., Hamm, R. J., Marmarou, A., et al. (1987). A fluid percussion model of experimental brain injury in the rat. J. Neurosurg. 67, 110–119. doi: 10.3171/jns.1987.67.1.0110
Dong, H., Zhang, Y., Huang, Y., and Deng, H. (2022). Pathophysiology of RAGE in inflammatory diseases. Front. Immunol. 13:931473. doi: 10.3389/fimmu.2022.931473
Dong, X. X., Wang, Y., and Qin, Z. H. (2009). Molecular mechanisms of excitotoxicity and their relevance to pathogenesis of neurodegenerative diseases. Acta Pharmacol. Sin. 30, 379–387. doi: 10.1038/aps.2009.24
Dong, Y. X., Zhang, H. Y., Li, H. Y., Liu, P. H., Sui, Y., and Sun, X. H. (2018). Association between Alzheimer’s disease pathogenesis and early demyelination and oligodendrocyte dysfunction. Neural Regen. Res. 13, 908–914. doi: 10.4103/1673-5374.232486
Donkin, J. J., and Vink, R. (2010). Mechanisms of cerebral edema in traumatic brain injury: therapeutic developments. Curr. Opin. Neurol. 23, 293–299. doi: 10.1097/WCO.0b013e328337f451
Droge, W. (2002). Free radicals in the physiological control of cell function. Physiol. Rev. 82, 47–95. doi: 10.1152/physrev.00018.2001
Dyck, S. M., and Karimi-Abdolrezaee, S. (2015). Chondroitin sulfate proteoglycans: Key modulators in the developing and pathologic central nervous system. Exp. Neurol. 269, 169–187. doi: 10.1016/j.expneurol.2015.04.006
Eakin, K., Rowe, R. K., and Lifshitz, J. (2015). “Modeling Fluid Percussion Injury: Relevance to Human Traumatic Brain Injury,” in Brain Neurotrauma: Molecular, Neuropsychological, and Rehabilitation Aspects, ed. F. H. Kobeissy (Boca Raton, FL: Taylor and Francis).
Early, A. N., Gorman, A. A., Van Eldik, L. J., Bachstetter, A. D., and Morganti, J. M. (2020). Effects of advanced age upon astrocyte-specific responses to acute traumatic brain injury in mice. J. Neuroinflammation 17:115. doi: 10.1186/s12974-020-01800-w
Edwards, K. A., Gill, J. M., Pattinson, C. L., Lai, C., Briere, M., Rogers, N. J., et al. (2020). Interleukin-6 is associated with acute concussion in military combat personnel. BMC Neurol. 20:209. doi: 10.1186/s12883-020-01760-x
Eikelenboom, P., van Exel, E., Hoozemans, J. J., Veerhuis, R., Rozemuller, A. J., and van Gool, W. A. (2010). Neuroinflammation - an early event in both the history and pathogenesis of Alzheimer’s disease. Neurodegener. Dis. 7, 38–41. doi: 10.1159/000283480
Escartin, C., Galea, E., Lakatos, A., O’Callaghan, J. P., Petzold, G. C., Serrano-Pozo, A., et al. (2021). Reactive astrocyte nomenclature, definitions, and future directions. Nat. Neurosci. 24, 312–325. doi: 10.1038/s41593-020-00783-4
Fan, H., Tang, H. B., Chen, Z., Wang, H. Q., Zhang, L., Jiang, Y., et al. (2020). Inhibiting HMGB1-RAGE axis prevents pro-inflammatory macrophages/microglia polarization and affords neuroprotection after spinal cord injury. J. Neuroinflammation 17:295. doi: 10.1186/s12974-020-01973-4
Fan, J., Fong, T., Chen, X., Chen, C., Luo, P., and Xie, H. (2018). Glia maturation factor-beta: a potential therapeutic target in neurodegeneration and neuroinflammation. Neuropsychiatr. Dis. Treat. 14, 495–504. doi: 10.2147/NDT.S157099
Fang, Y., and Eglen, R. M. (2017). Three-dimensional cell cultures in drug discovery and development. SLAS Discov 22, 456–472. doi: 10.1177/1087057117696795
Faul, M., and Coronado, V. (2015). Epidemiology of traumatic brain injury. Handb. Clin. Neurol. 127, 3–13. doi: 10.1016/B978-0-444-52892-6.00001-5
Fedor, M., Berman, R. F., Muizelaar, J. P., and Lyeth, B. G. (2010). Hippocampal theta dysfunction after lateral fluid percussion injury. J. Neurotrauma 27, 1605–1615. doi: 10.1089/neu.2010.1370
Feeney, D. M., Boyeson, M. G., Linn, R. T., Murray, H. M., and Dail, W. G. (1981). Responses to cortical injury: I. Methodology and local effects of contusions in the rat. Brain Res 211, 67–77. doi: 10.1016/0006-8993(81)90067-6
Feng, X., Jopson, T. D., Paladini, M. S., Liu, S., West, B. L., Gupta, N., et al. (2016). Colony-stimulating factor 1 receptor blockade prevents fractionated whole-brain irradiation-induced memory deficits. J. Neuroinflammation 13:215. doi: 10.1186/s12974-016-0671-y
Fernandez-Gajardo, R., Matamala, J. M., Carrasco, R., Gutierrez, R., Melo, R., and Rodrigo, R. (2014). Novel therapeutic strategies for traumatic brain injury: acute antioxidant reinforcement. CNS Drugs 28, 229–248. doi: 10.1007/s40263-013-0138-y
Fidan, E., Lewis, J., Kline, A. E., Garman, R. H., Alexander, H., Cheng, J. P., et al. (2016). Repetitive mild traumatic brain injury in the developing brain: effects on long-term functional outcome and neuropathology. J. Neurotrauma 33, 641–651. doi: 10.1089/neu.2015.3958
Fielder, E., von Zglinicki, T., and Jurk, D. (2017). The DNA damage response in neurons: Die by apoptosis or survive in a senescence-like state? J. Alzheimers Dis. 60, S107–S131. doi: 10.3233/JAD-161221
Filipi, T., Hermanova, Z., Tureckova, J., Vanatko, O., and Anderova, M. (2020). Glial Cells-The Strategic Targets in Amyotrophic Lateral Sclerosis Treatment. J. Clin. Med. 9:261. doi: 10.3390/jcm9010261
Filipi, T., Matusova, Z., Abaffy, P., Vanatko, O., Tureckova, J., Benesova, S., et al. (2023). Cortical glia in SOD1(G93A) mice are subtly affected by ALS-like pathology. Sci. Rep. 13:6538. doi: 10.1038/s41598-023-33608-y
Fisher, E. M. C., Greensmith, L., Malaspina, A., Fratta, P., Hanna, M. G., Schiavo, G., et al. (2023). Opinion: more mouse models and more translation needed for ALS. Mol Neurodegener. 18:30. doi: 10.1186/s13024-023-00619-2
Fitzgerald, J., Houle, S., Cotter, C., Zimomra, Z., Martens, K. M., Vonder Haar, C., et al. (2022). Lateral fluid percussion injury causes sex-specific deficits in anterograde but not retrograde memory. Front. Behav. Neurosci. 16:806598. doi: 10.3389/fnbeh.2022.806598
Fleminger, S., Oliver, D. L., Lovestone, S., Rabe-Hesketh, S., and Giora, A. (2003). Head injury as a risk factor for Alzheimer’s disease: the evidence 10 years on; a partial replication. J. Neurol. Neurosurg. Psychiatry 74, 857–862. doi: 10.1136/jnnp.74.7.857
Floyd, C. L., Golden, K. M., Black, R. T., Hamm, R. J., and Lyeth, B. G. (2002). Craniectomy position affects morris water maze performance and hippocampal cell loss after parasagittal fluid percussion. J. Neurotrauma 19, 303–316. doi: 10.1089/089771502753594873
Flygt, J., Gumucio, A., Ingelsson, M., Skoglund, K., Holm, J., Alafuzoff, I., et al. (2016). Human traumatic brain injury results in oligodendrocyte death and increases the number of oligodendrocyte progenitor cells. J. Neuropathol. Exp. Neurol. 75, 503–515. doi: 10.1093/jnen/nlw025
Flygt, J., Ruscher, K., Norberg, A., Mir, A., Gram, H., Clausen, F., et al. (2018). Neutralization of interleukin-1beta following diffuse traumatic brain injury in the mouse attenuates the loss of mature oligodendrocytes. J. Neurotrauma 35, 2837–2849. doi: 10.1089/neu.2018.5660
Fox, G. B., Fan, L., Levasseur, R. A., and Faden, A. I. (1998). Sustained sensory/motor and cognitive deficits with neuronal apoptosis following controlled cortical impact brain injury in the mouse. J. Neurotrauma 15, 599–614. doi: 10.1089/neu.1998.15.599
Fridovich, I. (2013). Oxygen: how do we stand it? Med. Princ. Pract. 22, 131–137. doi: 10.1159/000339212
Fronczak, K. M., Roberts, A., Svirsky, S., Parry, M., Holets, E., Henchir, J., et al. (2022). Assessment of behavioral, neuroinflammatory, and histological responses in a model of rat repetitive mild fluid percussion injury at 2 weeks post-injury. Front. Neurol. 13:945735. doi: 10.3389/fneur.2022.945735
Fujimoto, S. T., Longhi, L., Saatman, K. E., Conte, V., Stocchetti, N., and McIntosh, T. K. (2004). Motor and cognitive function evaluation following experimental traumatic brain injury. Neurosci. Biobehav. Rev 28, 365–378. doi: 10.1016/j.neubiorev.2004.06.002
Galgano, M., Toshkezi, G., Qiu, X., Russell, T., Chin, L., and Zhao, L. R. (2017). Traumatic brain injury: Current treatment strategies and future endeavors. Cell Transplant 26, 1118–1130. doi: 10.1177/0963689717714102
Gao, W., Guo, L., Yang, Y., Wang, Y., Xia, S., Gong, H., et al. (2021). Dissecting the crosstalk between Nrf2 and Nf-kappab response pathways in drug-induced toxicity. Front. Cell Dev. Biol. 9:809952. doi: 10.3389/fcell.2021.809952
Gao, Z., Zhu, Q., Zhang, Y., Zhao, Y., Cai, L., Shields, C. B., et al. (2013). Reciprocal modulation between microglia and astrocyte in reactive gliosis following the CNS injury. Mol. Neurobiol. 48, 690–701. doi: 10.1007/s12035-013-8460-4
Gardner, R. C., Byers, A. L., Barnes, D. E., Li, Y., Boscardin, J., and Yaffe, K. (2018). Mild TBI and risk of Parkinson disease: A chronic effects of neurotrauma consortium study. Neurology 90, e1771–e1779. doi: 10.1212/WNL.0000000000005522
Garza, R., Sharma, Y., Atacho, D. A. M., Thiruvalluvan, A., Abu Hamdeh, S., Jonsson, M. E., et al. (2023). Single-cell transcriptomics of human traumatic brain injury reveals activation of endogenous retroviruses in oligodendroglia. Cell Rep. 42:113395. doi: 10.1016/j.celrep.2023.113395
Gerzanich, V., Stokum, J. A., Ivanova, S., Woo, S. K., Tsymbalyuk, O., Sharma, A., et al. (2019). Sulfonylurea receptor 1, transient receptor potential cation channel subfamily m member 4, and KIR6.2: Role in hemorrhagic progression of contusion. J. Neurotrauma 36, 1060–1079. doi: 10.1089/neu.2018.5986
Goldman, S. M., Tanner, C. M., Oakes, D., Bhudhikanok, G. S., Gupta, A., and Langston, J. W. (2006). Head injury and Parkinson’s disease risk in twins. Ann. Neurol. 60, 65–72. doi: 10.1002/ana.20882
Goodrich, J. A., Kim, J. H., Situ, R., Taylor, W., Westmoreland, T., Du, F., et al. (2016). Neuronal and glial changes in the brain resulting from explosive blast in an experimental model. Acta Neuropathol. Commun. 4:124. doi: 10.1186/s40478-016-0395-3
Gorgoraptis, N., Zaw-Linn, J., Feeney, C., Tenorio-Jimenez, C., Niemi, M., Malik, A., et al. (2019). Cognitive impairment and health-related quality of life following traumatic brain injury. NeuroRehabilitation 44, 321–331. doi: 10.3233/NRE-182618
Grande, A., Sumiyoshi, K., Lopez-Juarez, A., Howard, J., Sakthivel, B., Aronow, B., et al. (2013). Environmental impact on direct neuronal reprogramming in vivo in the adult brain. Nat. Commun. 4:2373. doi: 10.1038/ncomms3373
Green, R. E., Colella, B., Maller, J. J., Bayley, M., Glazer, J., and Mikulis, D. J. (2014). Scale and pattern of atrophy in the chronic stages of moderate-severe TBI. Front. Hum. Neurosci. 8:67. doi: 10.3389/fnhum.2014.00067
Gu, D., Ou, S., and Liu, G. (2022). Traumatic brain injury and risk of dementia and Alzheimer’s disease: A systematic review and meta-analysis. Neuroepidemiology 56, 4–16. doi: 10.1159/000520966
Guan, B., Anderson, D. B., Chen, L., Feng, S., and Zhou, H. (2023). Global, regional and national burden of traumatic brain injury and spinal cord injury, 1990-2019: a systematic analysis for the Global Burden of Disease Study 2019. BMJ Open 13, e075049. doi: 10.1136/bmjopen-2023-075049
Guo, Z., Zhang, L., Wu, Z., Chen, Y., Wang, F., and Chen, G. (2014). In vivo direct reprogramming of reactive glial cells into functional neurons after brain injury and in an Alzheimer’s disease model. Cell Stem Cell 14, 188–202. doi: 10.1016/j.stem.2013.12.001
Gupte, R., Brooks, W., Vukas, R., Pierce, J., and Harris, J. (2019). Sex Differences in Traumatic Brain Injury: What We Know and What We Should Know. J. Neurotrauma 36, 3063–3091. doi: 10.1089/neu.2018.6171
Gyoneva, S., and Ransohoff, R. M. (2015). Inflammatory reaction after traumatic brain injury: therapeutic potential of targeting cell-cell communication by chemokines. Trends Pharmacol. Sci. 36, 471–480. doi: 10.1016/j.tips.2015.04.003
Hackett, A. R., Yahn, S. L., Lyapichev, K., Dajnoki, A., Lee, D. H., Rodriguez, M., et al. (2018). Injury type-dependent differentiation of NG2 glia into heterogeneous astrocytes. Exp. Neurol. 308, 72–79. doi: 10.1016/j.expneurol.2018.07.001
Hakiminia, B., Alikiaii, B., Khorvash, F., and Mousavi, S. (2022). Oxidative stress and mitochondrial dysfunction following traumatic brain injury: From mechanistic view to targeted therapeutic opportunities. Fundam Clin. Pharmacol. 36, 612–662. doi: 10.1111/fcp.12767
Hamm, R. J., Dixon, C. E., Gbadebo, D. M., Singha, A. K., Jenkins, L. W., Lyeth, B. G., et al. (1992). Cognitive deficits following traumatic brain injury produced by controlled cortical impact. J. Neurotrauma 9, 11–20. doi: 10.1089/neu.1992.9.11
Hamm, R. J., Pike, B. R., Odell, D. M., Lyeth, B. G., and Jenkins, L. W. (1994). The Rotarod Test - an Evaluation of Its Effectiveness in Assessing Motor Deficits Following Traumatic Brain Injury. J. Neurotrauma 11, 187–196. doi: 10.1089/neu.1994.11.187
Hassan, S. S. U., Samanta, S., Dash, R., Karpinski, T. M., Habibi, E., Sadiq, A., et al. (2022). The neuroprotective effects of fisetin, a natural flavonoid in neurodegenerative diseases: Focus on the role of oxidative stress. Front. Pharmacol. 13:1015835. doi: 10.3389/fphar.2022.1015835
Head, D., Buckner, R. L., Shimony, J. S., Williams, L. E., Akbudak, E., Conturo, T. E., et al. (2004). Differential vulnerability of anterior white matter in non-demented aging with minimal acceleration in dementia of the Alzheimer type: evidence from diffusion tensor imaging. Cereb. Cortex 14, 410–423. doi: 10.1093/cercor/bhh003
Heinrich, C., Bergami, M., Gascon, S., Lepier, A., Vigano, F., Dimou, L., et al. (2014). Sox2-mediated conversion of NG2 glia into induced neurons in the injured adult cerebral cortex. Stem Cell Rep. 3, 1000–1014. doi: 10.1016/j.stemcr.2014.10.007
Heinrich, C., Blum, R., Gascon, S., Masserdotti, G., Tripathi, P., Sanchez, R., et al. (2010). Directing astroglia from the cerebral cortex into subtype specific functional neurons. PLoS Biol. 8:e1000373. doi: 10.1371/journal.pbio.1000373
Henry, C. J., Huang, Y., Wynne, A. M., and Godbout, J. P. (2009). Peripheral lipopolysaccharide (LPS) challenge promotes microglial hyperactivity in aged mice that is associated with exaggerated induction of both pro-inflammatory IL-1beta and anti-inflammatory IL-10 cytokines. Brain Behav. Immun. 23, 309–317. doi: 10.1016/j.bbi.2008.09.002
Henry, R. J., Ritzel, R. M., Barrett, J. P., Doran, S. J., Jiao, Y., Leach, J. B., et al. (2020). Microglial Depletion with CSF1R Inhibitor During Chronic Phase of Experimental Traumatic Brain Injury Reduces Neurodegeneration and Neurological Deficits. J. Neurosci. 40, 2960–2974. doi: 10.1523/JNEUROSCI.2402-19.2020
Hentig, J., Cloghessy, K., Lahne, M., Jung, Y. J., Petersen, R. A., Morris, A. C., et al. (2021). Zebrafish Blunt-Force TBI induces heterogenous injury pathologies that mimic human TBI and responds with sonic hedgehog-dependent cell proliferation across the neuroaxis. Biomedicines 9:861. doi: 10.3390/biomedicines9080861
Hernandez, A., Tan, C., Plattner, F., Logsdon, A. F., Pozo, K., Yousuf, M. A., et al. (2018). Exposure to mild blast forces induces neuropathological effects, neurophysiological deficits and biochemical changes. Mol. Brain 11:64. doi: 10.1186/s13041-018-0408-1
Hernandez, I. H., Villa-Gonzalez, M., Martin, G., Soto, M., and Perez-Alvarez, M. J. (2021). Glial cells as therapeutic approaches in brain ischemia-reperfusion injury. Cells 10:1639. doi: 10.3390/cells10071639
Hiebert, J. B., Shen, Q., Thimmesch, A. R., and Pierce, J. D. (2015). Traumatic brain injury and mitochondrial dysfunction. Am. J. Med. Sci. 350, 132–138. doi: 10.1097/MAJ.0000000000000506
Higgins, G. C., Beart, P. M., Shin, Y. S., Chen, M. J., Cheung, N. S., and Nagley, P. (2010). Oxidative stress: emerging mitochondrial and cellular themes and variations in neuronal injury. J. Alzheimers Dis. 20, S453–S473. doi: 10.3233/JAD-2010-100321
Hill, R. A., Patel, K. D., Goncalves, C. M., Grutzendler, J., and Nishiyama, A. (2014). Modulation of oligodendrocyte generation during a critical temporal window after NG2 cell division. Nat. Neurosci. 17, 1518–1527. doi: 10.1038/nn.3815
Hiskens, M. I., Angoa-Perez, M., Schneiders, A. G., Vella, R. K., and Fenning, A. S. (2019). Modeling sports-related mild traumatic brain injury in animals-A systematic review. J. Neurosci. Res. 97, 1194–1222. doi: 10.1002/jnr.24472
Hoffman, A. N., Watson, S. L., Makridis, A. S., Patel, A. Y., Gonzalez, S. T., Ferguson, L., et al. (2020). Sex Differences in Behavioral Sensitivities After Traumatic Brain Injury. Front. Neurol. 11:553190. doi: 10.3389/fneur.2020.553190
Honsa, P., Valny, M., Kriska, J., Matuskova, H., Harantova, L., Kirdajova, D., et al. (2016). Generation of reactive astrocytes from NG2 cells is regulated by sonic hedgehog. Glia 64, 1518–1531. doi: 10.1002/glia.23019
Hotta, N., Aoyama, M., Inagaki, M., Ishihara, M., Miura, Y., Tada, T., et al. (2005). Expression of glia maturation factor beta after cryogenic brain injury. Brain Res. Mol. Brain Res. 133, 71–77. doi: 10.1016/j.molbrainres.2004.09.027
Huber, B. R., Meabon, J. S., Hoffer, Z. S., Zhang, J., Hoekstra, J. G., Pagulayan, K. F., et al. (2016). Blast exposure causes dynamic microglial/macrophage responses and microdomains of brain microvessel dysfunction. Neuroscience 319, 206–220. doi: 10.1016/j.neuroscience.2016.01.022
Huber, B. R., Meabon, J. S., Martin, T. J., Mourad, P. D., Bennett, R., Kraemer, B. C., et al. (2013). Blast exposure causes early and persistent aberrant phospho- and cleaved-tau expression in a murine model of mild blast-induced traumatic brain injury. J. Alzheimers Dis. 37, 309–323. doi: 10.3233/JAD-130182
Hughes, E. G., Kang, S. H., Fukaya, M., and Bergles, D. E. (2013). Oligodendrocyte progenitors balance growth with self-repulsion to achieve homeostasis in the adult brain. Nat. Neurosci. 16, 668–676. doi: 10.1038/nn.3390
Hughes, E. G., Maguire, J. L., McMinn, M. T., Scholz, R. E., and Sutherland, M. L. (2004). Loss of glial fibrillary acidic protein results in decreased glutamate transport and inhibition of PKA-induced EAAT2 cell surface trafficking. Brain Res. Mol. Brain Res. 124, 114–123. doi: 10.1016/j.molbrainres.2004.02.021
Hussain, S. F., Raza, Z., Cash, A. T. G., Zampieri, T., Mazzoli, R. A., Kardon, R. H., et al. (2021). Traumatic brain injury and sight loss in military and veteran populations- a review. Mil. Med. Res. 8:42. doi: 10.1186/s40779-021-00334-3
Hyder, A. A., Wunderlich, C. A., Puvanachandra, P., Gururaj, G., and Kobusingye, O. C. (2007). The impact of traumatic brain injuries: a global perspective. NeuroRehabilitation 22, 341–353.
Iaccarino, C., Carretta, A., Nicolosi, F., and Morselli, C. (2018). Epidemiology of severe traumatic brain injury. J. Neurosurg Sci. 62, 535–541. doi: 10.23736/S0390-5616.18.04532-0
Igarashi, T., Potts, M. B., and Noble-Haeusslein, L. J. (2007). Injury severity determines Purkinje cell loss and microglial activation in the cerebellum after cortical contusion injury. Exp. Neurol. 203, 258–268. doi: 10.1016/j.expneurol.2006.08.030
Ikeda, K., Kundu, R. K., Ikeda, S., Kobara, M., Matsubara, H., and Quertermous, T. (2006). Glia maturation factor-gamma is preferentially expressed in microvascular endothelial and inflammatory cells and modulates actin cytoskeleton reorganization. Circ. Res. 99, 424–433. doi: 10.1161/01.RES.0000237662.23539.0b
Illarionova, N. B., Gunnarson, E., Li, Y., Brismar, H., Bondar, A., Zelenin, S., et al. (2010). Functional and molecular interactions between aquaporins and Na,K-ATPase. Neuroscience 168, 915–925. doi: 10.1016/j.neuroscience.2009.11.062
Irvine, K. A., and Blakemore, W. F. (2008). Remyelination protects axons from demyelination-associated axon degeneration. Brain 131, 1464–1477. doi: 10.1093/brain/awn080
Izzy, S., Liu, Q., Fang, Z., Lule, S., Wu, L., Chung, J. Y., et al. (2019). Time-dependent changes in microglia transcriptional networks following traumatic brain injury. Front. Cell Neurosci. 13:307. doi: 10.3389/fncel.2019.00307
Jafari, S., Etminan, M., Aminzadeh, F., and Samii, A. (2013). Head injury and risk of Parkinson disease: a systematic review and meta-analysis. Mov. Disord. 28, 1222–1229. doi: 10.1002/mds.25458
Jain, M., Singh, M. K., Shyam, H., Mishra, A., Kumar, S., Kumar, A., et al. (2021). Role of JAK/STAT in the neuroinflammation and its association with neurological disorders. Ann. Neurosci. 28, 191–200. doi: 10.1177/09727531211070532
Jain, S., and Iverson, L. M. (2022). “Glasgow coma scale,” in StatPearls. Available online at: https://www.ncbi.nlm.nih.gov/books/NBK513298/ (accessed June 21, 2022).
Jantti, H., Sitnikova, V., Ishchenko, Y., Shakirzyanova, A., Giudice, L., Ugidos, I. F., et al. (2022). Microglial amyloid beta clearance is driven by PIEZO1 channels. J. Neuroinflammation 19:147. doi: 10.1186/s12974-022-02486-y
Jassam, Y. N., Izzy, S., Whalen, M., McGavern, D. B., and El Khoury, J. (2017). Neuroimmunology of Traumatic Brain Injury: Time for a Paradigm Shift. Neuron 95, 1246–1265. doi: 10.1016/j.neuron.2017.07.010
Jayakumar, A. R., Panickar, K. S., Curtis, K. M., Tong, X. Y., Moriyama, M., and Norenberg, M. D. (2011). Na-K-Cl cotransporter-1 in the mechanism of cell swelling in cultured astrocytes after fluid percussion injury. J. Neurochem. 117, 437–448. doi: 10.1111/j.1471-4159.2011.07211.x
Jayakumar, A. R., Tong, X. Y., Ruiz-Cordero, R., Bregy, A., Bethea, J. R., Bramlett, H. M., et al. (2014). Activation of NF-kappaB mediates astrocyte swelling and brain edema in traumatic brain injury. J. Neurotrauma 31, 1249–1257. doi: 10.1089/neu.2013.3169
Jeter, C. B., Hergenroeder, G. W., Ward, N. H. III, Moore, A. N., and Dash, P. K. (2012). Human traumatic brain injury alters circulating L-arginine and its metabolite levels: possible link to cerebral blood flow, extracellular matrix remodeling, and energy status. J. Neurotrauma 29, 119–127. doi: 10.1089/neu.2011.2029
Jha, R. M., Bell, J., Citerio, G., Hemphill, J. C., Kimberly, W. T., Narayan, R. K., et al. (2020). Role of Sulfonylurea Receptor 1 and Glibenclamide in Traumatic Brain Injury: A Review of the Evidence. Int. J. Mol. Sci. 21:409. doi: 10.3390/ijms21020409
Jha, R. M., Rani, A., Desai, S. M., Raikwar, S., Mihaljevic, S., Munoz-Casabella, A., et al. (2021). Sulfonylurea Receptor 1 in Central Nervous System Injury: An Updated Review. Int. J. Mol. Sci. 22:11899. doi: 10.3390/ijms222111899
Jiang, H., Wang, Y., Liang, X., Xing, X., Xu, X., and Zhou, C. (2018). Toll-Like Receptor 4 Knockdown Attenuates Brain Damage and Neuroinflammation After Traumatic Brain Injury via Inhibiting Neuronal Autophagy and Astrocyte Activation. Cell Mol. Neurobiol. 38, 1009–1019. doi: 10.1007/s10571-017-0570-5
Johann, S., and Beyer, C. (2013). Neuroprotection by gonadal steroid hormones in acute brain damage requires cooperation with astroglia and microglia. J. Steroid. Biochem. Mol. Biol. 137, 71–81. doi: 10.1016/j.jsbmb.2012.11.006
Johnson, N. H., de Rivero Vaccari, J. P., Bramlett, H. M., Keane, R. W., and Dietrich, W. D. (2023). Inflammasome activation in traumatic brain injury and Alzheimer’s disease. Transl. Res. 254, 1–12. doi: 10.1016/j.trsl.2022.08.014
Johnson, V. E., Stewart, J. E., Begbie, F. D., Trojanowski, J. Q., Smith, D. H., and Stewart, W. (2013a). Inflammation and white matter degeneration persist for years after a single traumatic brain injury. Brain 136, 28–42. doi: 10.1093/brain/aws322
Johnson, V. E., Stewart, W., and Smith, D. H. (2013b). Axonal pathology in traumatic brain injury. Exp. Neurol. 246, 35–43. doi: 10.1016/j.expneurol.2012.01.013
Kalluri, R., and LeBleu, V. S. (2020). The biology, function, and biomedical applications of exosomes. Science 367:aau6977. doi: 10.1126/science.aau6977
Kang, S. H., Fukaya, M., Yang, J. K., Rothstein, J. D., and Bergles, D. E. (2010). NG2 + CNS glial progenitors remain committed to the oligodendrocyte lineage in postnatal life and following neurodegeneration. Neuron 68, 668–681. doi: 10.1016/j.neuron.2010.09.009
Kapoor, S., Kim, S. M., Farook, J. M., Mir, S., Saha, R., and Sen, N. (2013). Foxo3a transcriptionally upregulates AQP4 and induces cerebral edema following traumatic brain injury. J. Neurosci. 33, 17398–17403. doi: 10.1523/JNEUROSCI.2756-13.2013
Karlander, M., Ljungqvist, J., and Zelano, J. (2021). Post-traumatic epilepsy in adults: a nationwide register-based study. J. Neurol. Neurosurg. Psychiatry 92, 617–621. doi: 10.1136/jnnp-2020-325382
Karve, I. P., Taylor, J. M., and Crack, P. J. (2016). The contribution of astrocytes and microglia to traumatic brain injury. Br. J. Pharmacol. 173, 692–702. doi: 10.1111/bph.13125
Katzenberger, R. J., Loewen, C. A., Wassarman, D. R., Petersen, A. J., Ganetzky, B., and Wassarman, D. A. (2013). A Drosophila model of closed head traumatic brain injury. Proc. Natl. Acad. Sci. U. S. A. 110, E4152–E4159. doi: 10.1073/pnas.1316895110
Kelley, N., Jeltema, D., Duan, Y., and He, Y. (2019). The NLRP3 Inflammasome: An Overview of Mechanisms of Activation and Regulation. Int. J. Mol. Sci. 20, 3328. doi: 10.3390/ijms20133328
Kernie, S. G., Erwin, T. M., and Parada, L. F. (2001). Brain remodeling due to neuronal and astrocytic proliferation after controlled cortical injury in mice. J. Neurosci. Res. 66, 317–326. doi: 10.1002/jnr.10013
Khayatan, D., Razavi, S. M., Arab, Z. N., Niknejad, A. H., Nouri, K., Momtaz, S., et al. (2022). Protective effects of curcumin against traumatic brain injury. Biomed. Pharmacother. 154:113621. doi: 10.1016/j.biopha.2022.113621
Kim, Y., Park, J., and Choi, Y. K. (2019). The role of astrocytes in the central nervous system focused on BK channel and heme oxygenase metabolites: A review. Antioxidants 8:121. doi: 10.3390/antiox8050121
Kim, Y. S., Jung, H. M., and Yoon, B. E. (2018). Exploring glia to better understand Alzheimer’s disease. Anim. Cells Syst. 22, 213–218. doi: 10.1080/19768354.2018.1508498
King, Z. A., Sheth, K. N., Kimberly, W. T., and Simard, J. M. (2018). Profile of intravenous glyburide for the prevention of cerebral edema following large hemispheric infarction: evidence to date. Drug Des. Devel Ther. 12, 2539–2552. doi: 10.2147/DDDT.S150043
Kinney, J. W., Bemiller, S. M., Murtishaw, A. S., Leisgang, A. M., Salazar, A. M., and Lamb, B. T. (2018). Inflammation as a central mechanism in Alzheimer’s disease. Alzheimers Dement. 4, 575–590. doi: 10.1016/j.trci.2018.06.014
Kitchen, P., Salman, M. M., Halsey, A. M., Clarke-Bland, C., MacDonald, J. A., Ishida, H., et al. (2020). Targeting Aquaporin-4 Subcellular Localization to Treat Central Nervous System Edema. Cell 78, e719. doi: 10.1016/j.cell.2020.03.037
Kochanek, P. M., Wallisch, J. S., Bayir, H., and Clark, R. S. B. (2017). Pre-clinical models in pediatric traumatic brain injury-challenges and lessons learned. Childs Nerv. Syst. 33, 1693–1701. doi: 10.1007/s00381-017-3474-2
Kodali, M., Madhu, L. N., Reger, R. L., Milutinovic, B., Upadhya, R., Gonzalez, J. J., et al. (2023). Intranasally administered human MSC-derived extracellular vesicles inhibit NLRP3-p38/MAPK signaling after TBI and prevent chronic brain dysfunction. Brain Behav. Immun. 108, 118–134. doi: 10.1016/j.bbi.2022.11.014
Kofler, B., Bulleyment, A., Humphries, A., and Carter, D. A. (2002). Id-1 expression defines a subset of vimentin/S-100beta-positive. GFAP-negative astrocytes in the adult rat pineal gland. Histochem. J. 34, 167–171. doi: 10.1023/a:1020946631937
Kohanbash, G., and Okada, H. (2012). MicroRNAs and STAT interplay. Semin. Cancer Biol. 22, 70–75. doi: 10.1016/j.semcancer.2011.12.010
Kong, L. Z., Zhang, R. L., Hu, S. H., and Lai, J. B. (2022). Military traumatic brain injury: a challenge straddling neurology and psychiatry. Mil. Med. Res. 9:2. doi: 10.1186/s40779-021-00363-y
Korbecki, J., Bobinski, R., and Dutka, M. (2019). Self-regulation of the inflammatory response by peroxisome proliferator-activated receptors. Inflamm. Res. 68, 443–458. doi: 10.1007/s00011-019-01231-1
Kosari-Nasab, M., Shokouhi, G., Ghorbanihaghjo, A., Mesgari-Abbasi, M., and Salari, A. A. (2019). Quercetin mitigates anxiety-like behavior and normalizes hypothalamus-pituitary-adrenal axis function in a mouse model of mild traumatic brain injury. Behav. Pharmacol. 30, 282–289. doi: 10.1097/FBP.0000000000000480
Kovac, S., Angelova, P. R., Holmstrom, K. M., Zhang, Y., Dinkova-Kostova, A. T., and Abramov, A. Y. (2015). Nrf2 regulates ROS production by mitochondria and NADPH oxidase. Biochim. Biophys. Acta 1850, 794–801. doi: 10.1016/j.bbagen.2014.11.021
Kramer-Albers, E. M., Bretz, N., Tenzer, S., Winterstein, C., Mobius, W., Berger, H., et al. (2007). Oligodendrocytes secrete exosomes containing major myelin and stress-protective proteins: Trophic support for axons? Proteomics Clin. Appl. 1, 1446–1461. doi: 10.1002/prca.200700522
Krieg, J. L., Leonard, A. V., Tuner, R. J., and Corrigan, F. (2023). Characterization of Traumatic Brain Injury in a Gyrencephalic Ferret Model Using the Novel Closed Head Injury Model of Engineered Rotational Acceleration (CHIMERA). Neurotrauma Rep. 4, 761–780. doi: 10.1089/neur.2023.0047
Kritsilis, M. S. V. R., Koutsoudaki, P. N., Evangelou, K., Gorgoulis, V. G., and Papadopoulos, D. (2018). Ageing, Cellular Senescence and Neurodegenerative Disease. Int. J. Mol. Sci. 19:2937. doi: 10.3390/ijms19102937
Kumar, A., Stoica, B. A., Loane, D. J., Yang, M., Abulwerdi, G., Khan, N., et al. (2017). Microglial-derived microparticles mediate neuroinflammation after traumatic brain injury. J. Neuroinflammation 14:47. doi: 10.1186/s12974-017-0819-4
Kumar, A., Stoica, B. A., Sabirzhanov, B., Burns, M. P., Faden, A. I., and Loane, D. J. (2013). Traumatic brain injury in aged animals increases lesion size and chronically alters microglial/macrophage classical and alternative activation states. Neurobiol. Aging 34, 1397–1411. doi: 10.1016/j.neurobiolaging.2012.11.013
Kumar, R. G., Diamond, M. L., Boles, J. A., Berger, R. P., Tisherman, S. A., Kochanek, P. M., et al. (2015). Acute CSF interleukin-6 trajectories after TBI: associations with neuroinflammation, polytrauma, and outcome. Brain Behav. Immun. 45, 253–262. doi: 10.1016/j.bbi.2014.12.021
Kumari, M., Arora, P., Sharma, P., Hasija, Y., Rana, P., D’Souza, M. M., et al. (2023). Acute metabolic alterations in the hippocampus are associated with decreased acetylation after blast induced TBI. Metabolomics 19:5. doi: 10.1007/s11306-022-01970-z
Laird, M. D., Shields, J. S., Sukumari-Ramesh, S., Kimbler, D. E., Fessler, R. D., Shakir, B., et al. (2014). High mobility group box protein-1 promotes cerebral edema after traumatic brain injury via activation of toll-like receptor 4. Glia 62, 26–38. doi: 10.1002/glia.22581
Lan, Y. L., Wang, X., Zou, Y. J., Xing, J. S., Lou, J. C., Zou, S., et al. (2019). Bazedoxifene protects cerebral autoregulation after traumatic brain injury and attenuates impairments in blood-brain barrier damage: involvement of anti-inflammatory pathways by blocking MAPK signaling. Inflamm. Res. 68, 311–323. doi: 10.1007/s00011-019-01217-z
Lang, B., Liu, H. L., Liu, R., Feng, G. D., Jiao, X. Y., and Ju, G. (2004). Astrocytes in injured adult rat spinal cord may acquire the potential of neural stem cells. Neuroscience 128, 775–783. doi: 10.1016/j.neuroscience.2004.06.033
Langlois, J. A., Rutland-Brown, W., and Wald, M. M. (2006). The epidemiology and impact of traumatic brain injury: a brief overview. J. Head Trauma Rehabil. 21, 375–378. doi: 10.1097/00001199-200609000-00001
LaPak, K. M., and Burd, C. E. (2014). The molecular balancing act of p16(INK4a) in cancer and aging. Mol. Cancer Res. 12, 167–183. doi: 10.1158/1541-7786.MCR-13-0350
Lee, C. Y., and Landreth, G. E. (2010). The role of microglia in amyloid clearance from the AD brain. J. Neural Transm. 117, 949–960. doi: 10.1007/s00702-010-0433-4
Lehmann, C., Bette, S., and Engele, J. (2009). High extracellular glutamate modulates expression of glutamate transporters and glutamine synthetase in cultured astrocytes. Brain Res. 1297, 1–8. doi: 10.1016/j.brainres.2009.08.070
Lei, P., Li, Y., Chen, X., Yang, S., and Zhang, J. (2009). Microarray based analysis of microRNA expression in rat cerebral cortex after traumatic brain injury. Brain Res. 1284, 191–201. doi: 10.1016/j.brainres.2009.05.074
Leng, Y., Byers, A. L., Barnes, D. E., Peltz, C. B., Li, Y., and Yaffe, K. (2021). Traumatic Brain Injury and Incidence Risk of Sleep Disorders in Nearly 200,000 US Veterans. Neurology 96, e1792–e1799. doi: 10.1212/WNL.0000000000011656
Leo, P., and McCrea, M. (2016). “Epidemiology,” in Translational Research in Traumatic Brain Injury, eds D. Laskowitz and G. Grant (Boca Raton, FL: Taylor and Francis).
Lerouet, D., Marchand-Leroux, C., and Besson, V. C. (2021). Neuropharmacology in traumatic brain injury: from preclinical to clinical neuroprotection? Fundam. Clin. Pharmacol. 35, 524–538. doi: 10.1111/fcp.12656
Levine, J. M. (1994). Increased expression of the NG2 chondroitin-sulfate proteoglycan after brain injury. J. Neurosci. 14, 4716–4730. doi: 10.1523/JNEUROSCI.14-08-04716.1994
Lewen, A., Matz, P., and Chan, P. H. (2000). Free radical pathways in CNS injury. J. Neurotrauma 17, 871–890. doi: 10.1089/neu.2000.17.871
Li, D., Huang, B., Liu, J., Li, L., and Li, X. (2013). Decreased brain K(ATP) channel contributes to exacerbating ischemic brain injury and the failure of neuroprotection by sevoflurane post-conditioning in diabetic rats. PLoS One 8:e73334. doi: 10.1371/journal.pone.0073334
Li, G., Duan, L., Yang, F., Yang, L., Deng, Y., Yu, Y., et al. (2022a). Curcumin suppress inflammatory response in traumatic brain injury via p38/MAPK signaling pathway. Phytother. Res. 36, 1326–1337. doi: 10.1002/ptr.7391
Li, Y. F., Ren, X., Zhang, L., Wang, Y. H., and Chen, T. (2022b). Microglial polarization in TBI: Signaling pathways and influencing pharmaceuticals. Front. Aging Neurosci. 14:901117. doi: 10.3389/fnagi.2022.901117
Li, W., He, Y., Zhang, R., Zheng, G., and Zhou, D. (2019). The curcumin analog EF24 is a novel senolytic agent. Aging 11, 771–782. doi: 10.18632/aging.101787
Li, X., Wang, H., Gao, Y., Li, L., Tang, C., Wen, G., et al. (2016). Protective Effects of Quercetin on Mitochondrial Biogenesis in Experimental Traumatic Brain Injury via the Nrf2 Signaling Pathway. PLoS One 11:e0164237. doi: 10.1371/journal.pone.0164237
Li, Y., Li, Y., Li, X., Zhang, S., Zhao, J., Zhu, X., et al. (2017). Head Injury as a Risk Factor for Dementia and Alzheimer’s Disease: A Systematic Review and Meta-Analysis of 32 Observational Studies. PLoS One 12:e0169650. doi: 10.1371/journal.pone.0169650
Lian, L., Liu, M., Cui, L., Guan, Y., Liu, T., Cui, B., et al. (2019). Environmental risk factors and amyotrophic lateral sclerosis (ALS): A case-control study of ALS in China. J. Clin. Neurosci. 66, 12–18. doi: 10.1016/j.jocn.2019.05.036
Liddelow, S. A., and Barres, B. A. (2017). Reactive astrocytes: Production, function, and therapeutic potential. Immunity 46, 957–967. doi: 10.1016/j.immuni.2017.06.006
Liddelow, S. A., Guttenplan, K. A., Clarke, L. E., Bennett, F. C., Bohlen, C. J., Schirmer, L., et al. (2017). Neurotoxic reactive astrocytes are induced by activated microglia. Nature 541, 481–487. doi: 10.1038/nature21029
Lim, R., and Zaheer, A. (1996). In vitro enhancement of p38 mitogen-activated protein kinase activity by phosphorylated glia maturation factor. J. Biol. Chem. 271, 22953–22956. doi: 10.1074/jbc.271.38.22953
Limbad, C., Oron, T. R., Alimirah, F., Davalos, A. R., Tracy, T. E., Gan, L., et al. (2020). Astrocyte senescence promotes glutamate toxicity in cortical neurons. PLoS One 15:e0227887. doi: 10.1371/journal.pone.0227887
Lindner, M. D., Plone, M. A., Cain, C. K., Frydel, B., Francis, J. M., Emerich, D. F., et al. (1998). Dissociable long-term cognitive deficits after frontal versus sensorimotor cortical contusions. J. Neurotrauma 15, 199–216. doi: 10.1089/neu.1998.15.199
Litwiniuk, A., Juszczak, G. R., Stankiewicz, A. M., and Urbanska, K. (2023). The role of glial autophagy in Alzheimer’s disease. Mol. Psychiatry doi: 10.1038/s41380-023-02242-5 [Epub ahead of print].
Liu, Y., Miao, Q., Yuan, J., Han, S., Zhang, P., Li, S., et al. (2015). Ascl1 Converts Dorsal Midbrain Astrocytes into Functional Neurons In Vivo. J. Neurosci. 35, 9336–9355. doi: 10.1523/JNEUROSCI.3975-14.2015
Loane, D. J., Kumar, A., Stoica, B. A., Cabatbat, R., and Faden, A. I. (2014). Progressive neurodegeneration after experimental brain trauma: association with chronic microglial activation. J. Neuropathol. Exp. Neurol. 73, 14–29. doi: 10.1097/NEN.0000000000000021
Loboda, A., Damulewicz, M., Pyza, E., Jozkowicz, A., and Dulak, J. (2016). Role of Nrf2/HO-1 system in development, oxidative stress response and diseases: an evolutionarily conserved mechanism. Cell Mol. Life Sci. 73, 3221–3247. doi: 10.1007/s00018-016-2223-0
Logsdon, A. F., Meabon, J. S., Cline, M. M., Bullock, K. M., Raskind, M. A., Peskind, E. R., et al. (2018). Blast exposure elicits blood-brain barrier disruption and repair mediated by tight junction integrity and nitric oxide dependent processes. Sci. Rep. 8:11344. doi: 10.1038/s41598-018-29341-6
Logsdon, A. F., Schindler, A. G., Meabon, J. S., Yagi, M., Herbert, M. J., Banks, W. A., et al. (2020). Nitric oxide synthase mediates cerebellar dysfunction in mice exposed to repetitive blast-induced mild traumatic brain injury. Sci. Rep. 10:9420. doi: 10.1038/s41598-020-66113-7
Logsdon, A. F., Turner, R. C., Lucke-Wold, B. P., Robson, M. J., Naser, Z. J., Smith, K. E., et al. (2014). Altering endoplasmic reticulum stress in a model of blast-induced traumatic brain injury controls cellular fate and ameliorates neuropsychiatric symptoms. Front. Cell Neurosci. 8:421. doi: 10.3389/fncel.2014.00421
Long, J. B., Gordon, J., Bettencourt, J. A., and Bolt, S. L. (1996). Laser-Doppler flowmetry measurements of subcortical blood flow changes after fluid percussion brain injury in rats. J. Neurotrauma 13, 149–162. doi: 10.1089/neu.1996.13.149
Lotocki, G., de Rivero Vaccari, J., Alonso, O., Molano, J. S., Nixon, R., Dietrich, W. D., et al. (2011). Oligodendrocyte Vulnerability Following Traumatic Brain Injury in Rats: Effect of Moderate Hypothermia. Ther. Hypothermia Temp. Manag. 1, 43–51. doi: 10.1089/ther.2010.0011
Lu, Y. C., Liu, S., Gong, Q. Z., Hamm, R. J., and Lyeth, B. G. (1997). Inhibition of nitric oxide synthase potentiates hypertension and increases mortality in traumatically brain-injured rats. Mol. Chem. Neuropathol. 30, 125–137. doi: 10.1007/BF02815154
Lucci, E. B. (2006). Civilian preparedness and counter-terrorism: conventional weapons. Surg. Clin. North Am. 86, 579–600. doi: 10.1016/j.suc.2006.03.001
Lund, H., Pieber, M., Parsa, R., Han, J., Grommisch, D., Ewing, E., et al. (2018). Competitive repopulation of an empty microglial niche yields functionally distinct subsets of microglia-like cells. Nat. Commun. 9:4845. doi: 10.1038/s41467-018-07295-7
Lund, S. B., Gjeilo, K. H., Moen, K. G., Schirmer-Mikalsen, K., Skandsen, T., and Vik, A. (2016). Moderate traumatic brain injury, acute phase course and deviations in physiological variables: an observational study. Scand. J. Trauma Resusc. Emerg. Med. 24:77. doi: 10.1186/s13049-016-0269-5
Luo, J., Nguyen, A., Villeda, S., Zhang, H., Ding, Z., Lindsey, D., et al. (2014). Long-term cognitive impairments and pathological alterations in a mouse model of repetitive mild traumatic brain injury. Front. Neurol. 5:12. doi: 10.3389/fneur.2014.00012
Ma, X., Aravind, A., Pfister, B. J., Chandra, N., and Haorah, J. (2019). Animal models of traumatic brain injury and assessment of injury severity. Mol. Neurobiol. 56, 5332–5345. doi: 10.1007/s12035-018-1454-5
Madan, S., Kron, B., Jin, Z., Al Shamy, G., Campeau, P. M., Sun, Q., et al. (2018). Arginase overexpression in neurons and its effect on traumatic brain injury. Mol. Genet. Metab. 125, 112–117. doi: 10.1016/j.ymgme.2018.07.007
Mader, M. M., and Czorlich, P. (2022). The role of L-arginine metabolism in neurocritical care patients. Neural Regen. Res. 17, 1446–1453. doi: 10.4103/1673-5374.327331
Madhok, D. Y., Rodriguez, R. M., Barber, J., Temkin, N. R., Markowitz, A. J., Kreitzer, N., et al. (2022). Outcomes in patients with mild traumatic brain injury without acute intracranial traumatic injury. JAMA Netw Open 5:e2223245. doi: 10.1001/jamanetworkopen.2022.23245
Mahoney, S. O., Chowdhury, N. F., Ngo, V., Imms, P., and Irimia, A. (2022). Mild traumatic brain injury results in significant and lasting cortical demyelination. Front. Neurol. 13:854396. doi: 10.3389/fneur.2022.854396
Manivannan, S., Wales, E., and Zaben, M. (2021). The Role of HMGB1 in Traumatic Brain Injury-Bridging the Gap Between the Laboratory and Clinical Studies. Curr. Neurol. Neurosci. Rep. 21:75. doi: 10.1007/s11910-021-01158-3
Maragakis, N. J., and Rothstein, J. D. (2006). Mechanisms of Disease: astrocytes in neurodegenerative disease. Nat. Clin. Pract. Neurol. 2, 679–689. doi: 10.1038/ncpneuro0355
Margulies, S. S., and Thibault, K. L. (2000). Infant skull and suture properties: measurements and implications for mechanisms of pediatric brain injury. J. Biomech. Eng. 122, 364–371. doi: 10.1115/1.1287160
Marmarou, A., Foda, M. A., van den Brink, W., Campbell, J., Kita, H., and Demetriadou, K. (1994). A new model of diffuse brain injury in rats. Part I: Pathophysiology and biomechanics. J. Neurosurg. 80, 291–300. doi: 10.3171/jns.1994.80.2.0291
Marmarou, A., Signoretti, S., Fatouros, P. P., Portella, G., Aygok, G. A., and Bullock, M. R. (2006). Predominance of cellular edema in traumatic brain swelling in patients with severe head injuries. J. Neurosurg. 104, 720–730. doi: 10.3171/jns.2006.104.5.720
Martinez-Coria, H., Arrieta-Cruz, I., Gutierrez-Juarez, R., and Lopez-Valdes, H. E. (2023). Anti-inflammatory effects of flavonoids in common neurological disorders associated with aging. Int. J. Mol. Sci. 24:4297. doi: 10.3390/ijms24054297
Martinez-Valverde, T., Vidal-Jorge, M., Martinez-Saez, E., Castro, L., Arikan, F., Cordero, E., et al. (2015). Sulfonylurea receptor 1 in humans with post-traumatic brain contusions. J. Neurotrauma 32, 1478–1487. doi: 10.1089/neu.2014.3706
Matias, I., Morgado, J., and Gomes, F. C. A. (2019). Astrocyte Heterogeneity: Impact to Brain Aging and Disease. Front. Aging Neurosci. 11:59. doi: 10.3389/fnagi.2019.00059
Mattugini, N., Merl-Pham, J., Petrozziello, E., Schindler, L., Bernhagen, J., Hauck, S. M., et al. (2018). Influence of white matter injury on gray matter reactive gliosis upon stab wound in the adult murine cerebral cortex. Glia 66, 1644–1662. doi: 10.1002/glia.23329
Mautes, A. E., Fukuda, K., and Noble, L. J. (1996). Cellular response in the cerebellum after midline traumatic brain injury in the rat. Neurosci. Lett. 214, 95–98. doi: 10.1016/0304-3940(96)12916-5
Mayer, S., Khakipoor, S., Dromer, M., and Cozetto, D. (2019). Single-cell RNA-Sequencing in Neuroscience. Neuroforum 25, 251–258. doi: 10.1515/nf-2019-0021
McDonald, B. Z., Gee, C. C., and Kievit, F. M. (2021). The nanotheranostic researcher’s guide for use of animal models of traumatic brain injury. J. Nanotheranostics 2, 224–268. doi: 10.3390/jnt2040014
McKee, A. C., Alosco, M. L., and Huber, B. R. (2016). Repetitive Head Impacts and Chronic Traumatic Encephalopathy. Neurosurg. Clin. N. Am. 27, 529–535. doi: 10.1016/j.nec.2016.05.009
McKee, A. C., Cantu, R. C., Nowinski, C. J., Hedley-Whyte, E. T., Gavett, B. E., Budson, A. E., et al. (2009). Chronic traumatic encephalopathy in athletes: progressive tauopathy after repetitive head injury. J. Neuropathol. Exp. Neurol. 68, 709–735. doi: 10.1097/NEN.0b013e3181a9d503
McKee, A. C., Daneshvar, D. H., Alvarez, V. E., and Stein, T. D. (2014). The neuropathology of sport. Acta Neuropathol. 127, 29–51. doi: 10.1007/s00401-013-1230-6
McKee, A. C., and Robinson, M. E. (2014). Military-related traumatic brain injury and neurodegeneration. Alzheimers Dement. 3, S242–S253. doi: 10.1016/j.jalz.2014.04.003
McNamara, E. H., Grillakis, A. A., Tucker, L. B., and McCabe, J. T. (2020). The closed-head impact model of engineered rotational acceleration (CHIMERA) as an application for traumatic brain injury pre-clinical research: A status report. Exp. Neurol. 333:113409. doi: 10.1016/j.expneurol.2020.113409
Mendes Arent, A., de Souza, L. F., Walz, R., and Dafre, A. L. (2014). Perspectives on molecular biomarkers of oxidative stress and antioxidant strategies in traumatic brain injury. Biomed. Res. Int. 2014:723060. doi: 10.1155/2014/723060
Menzies, F. M., Henriquez, F. L., Alexander, J., and Roberts, C. W. (2011). Selective inhibition and augmentation of alternative macrophage activation by progesterone. Immunology 134, 281–291. doi: 10.1111/j.1365-2567.2011.03488.x
Mesa Suarez, P., Santotoribio, J. D., Ramos Ramos, V., Gonzalez Garcia, M. A., Perez Ramos, S., Portilla Huertas, D., et al. (2016). [Brain damage after general anesthesia]. Med. Clin. 146, 384–388. doi: 10.1016/j.medcli.2016.01.018
Michinaga, S., and Koyama, Y. (2021). Pathophysiological Responses and Roles of Astrocytes in Traumatic Brain Injury. Int. J. Mol. Sci. 22:6418. doi: 10.3390/ijms22126418
Mielke, M. M., Ransom, J. E., Mandrekar, J., Turcano, P., Savica, R., and Brown, A. W. (2022). Traumatic Brain Injury and Risk of Alzheimer’s Disease and Related Dementias in the Population. J. Alzheimers Dis. 88, 1049–1059. doi: 10.3233/JAD-220159
Miller, G. F., DePadilla, L., and Xu, L. (2021). Costs of non-fatal traumatic brain injury in the United States, 2016. Med. Care 59, 451–455. doi: 10.1097/MLR.0000000000001511
Mills, C. D., Kincaid, K., Alt, J. M., Heilman, M. J., and Hill, A. M. (2000). M-1/M-2 macrophages and the Th1/Th2 paradigm. J. Immunol. 164, 6166–6173. doi: 10.4049/jimmunol.164.12.6166
Mira, R. G., Lira, M., and Cerpa, W. (2021). Traumatic Brain Injury: Mechanisms of Glial Response. Front. Physiol. 12:740939. doi: 10.3389/fphys.2021.740939
Miron, V. E., Boyd, A., Zhao, J. W., Yuen, T. J., Ruckh, J. M., Shadrach, J. L., et al. (2013). M2 microglia and macrophages drive oligodendrocyte differentiation during CNS remyelination. Nat. Neurosci. 16, 1211–1218. doi: 10.1038/nn.3469
Mishra, V., Skotak, M., Schuetz, H., Heller, A., Haorah, J., and Chandra, N. (2016). Primary blast causes mild, moderate, severe and lethal TBI with increasing blast overpressures: Experimental rat injury model. Sci. Rep. 6:26992. doi: 10.1038/srep26992
Miyamoto, A., Wake, H., Moorhouse, A. J., and Nabekura, J. (2013). Microglia and synapse interactions: fine tuning neural circuits and candidate molecules. Front. Cell Neurosci. 7:70. doi: 10.3389/fncel.2013.00070
Miyazawa, N., Diksic, M., and Yamamoto, Y. (1995). Chronological study of peripheral benzodiazepine binding sites in the rat brain stab wounds using [3H] PK-11195 as a marker for gliosis. Acta Neurochir. 137, 207–216. doi: 10.1007/BF02187195
Morgan, M. J., and Liu, Z. G. (2011). Crosstalk of reactive oxygen species and NF-kappaB signaling. Cell Res. 21, 103–115. doi: 10.1038/cr.2010.178
Morganti, J. M., Goulding, D. S., and Van Eldik, L. J. (2019). Deletion of p38alpha MAPK in microglia blunts trauma-induced inflammatory responses in mice. J. Neuroinflammation 16:98. doi: 10.1186/s12974-019-1493-5
Mori, T., Wang, X., Jung, J. C., Sumii, T., Singhal, A. B., Fini, M. E., et al. (2002). Mitogen-activated protein kinase inhibition in traumatic brain injury: in vitro and in vivo effects. J. Cereb. Blood Flow Metab. 22, 444–452. doi: 10.1097/00004647-200204000-00008
Morita, A., Jullienne, A., Salehi, A., Hamer, M., Javadi, E., Alsarraj, Y., et al. (2020). Temporal evolution of heme oxygenase-1 expression in reactive astrocytes and microglia in response to traumatic brain injury. Brain Hemorrhages 1, 65–74. doi: 10.1016/j.hest.2020.01.002
Moro, N., Ghavim, S. S., and Sutton, R. L. (2021). Massive efflux of adenosine triphosphate into the extracellular space immediately after experimental traumatic brain injury. Exp. Ther. Med. 21:575. doi: 10.3892/etm.2021.10007
Morris, R. (1984). Developments of a water-maze procedure for studying spatial learning in the rat. J. Neurosci. Methods 11, 47–60. doi: 10.1016/0165-0270(84)90007-4
Morrison, G., Fraser, D. D., and Cepinskas, G. (2013). Mechanisms and consequences of acquired brain injury during development. Pathophysiology 20, 49–57. doi: 10.1016/j.pathophys.2012.02.006
Mukherjee, S., Arisi, G. M., Mims, K., Hollingsworth, G., O’Neil, K., and Shapiro, L. A. (2020). Neuroinflammatory mechanisms of post-traumatic epilepsy. J. Neuroinflammation 17:193. doi: 10.1186/s12974-020-01854-w
Murray, H. C., Osterman, C., Bell, P., Vinnell, L., and Curtis, M. A. (2022). Neuropathology in chronic traumatic encephalopathy: a systematic review of comparative post-mortem histology literature. Acta Neuropathol. Commun. 10:108. doi: 10.1186/s40478-022-01413-9
Musi, N., Valentine, J. M., Sickora, K. R., Baeuerle, E., Thompson, C. S., Shen, Q., et al. (2018). Tau protein aggregation is associated with cellular senescence in the brain. Aging Cell. 17, e12840. doi: 10.1111/acel.12840
Mychasiuk, R., Hehar, H., Candy, S., Ma, I., and Esser, M. J. (2016). The direction of the acceleration and rotational forces associated with mild traumatic brain injury in rodents effect behavioural and molecular outcomes. J. Neurosci. Methods 257, 168–178. doi: 10.1016/j.jneumeth.2015.10.002
Nakano, M., Tamura, Y., Yamato, M., Kume, S., Eguchi, A., Takata, K., et al. (2017). NG2 glial cells regulate neuroimmunological responses to maintain neuronal function and survival. Sci. Rep. 7:42041. doi: 10.1038/srep42041
Namjoshi, D. R., Cheng, W. H., McInnes, K. A., Martens, K. M., Carr, M., Wilkinson, A., et al. (2014). Merging pathology with biomechanics using CHIMERA (Closed-Head Impact Model of Engineered Rotational Acceleration): a novel, surgery-free model of traumatic brain injury. Mol. Neurodegener. 9:55. doi: 10.1186/1750-1326-9-55
Nascimento, G. C., Bortolanza, M., Bribian, A., Leal-Luiz, G. C., Raisman-Vozari, R., Lopez-Mascaraque, L., et al. (2023). Dynamic Involvement of Striatal NG2-glia in L-DOPA Induced Dyskinesia in Parkinsonian Rats: Effects of Doxycycline. ASN Neuro. 15:17590914231155976. doi: 10.1177/17590914231155976
Natale, J. E., Ahmed, F., Cernak, I., Stoica, B., and Faden, A. I. (2003). Gene expression profile changes are commonly modulated across models and species after traumatic brain injury. J. Neurotrauma 20, 907–927. doi: 10.1089/089771503770195777
Neely, J. D., Amiry-Moghaddam, M., Ottersen, O. P., Froehner, S. C., Agre, P., and Adams, M. E. (2001). Syntrophin-dependent expression and localization of Aquaporin-4 water channel protein. Proc. Natl. Acad. Sci. U. S. A. 98, 14108–14113. doi: 10.1073/pnas.241508198
Network, B. I. C. C. (2021). A multimodal cell census and atlas of the mammalian primary motor cortex. Nature 598, 86–102. doi: 10.1038/s41586-021-03950-0
Neusch, C., Bahr, M., and Schneider-Gold, C. (2007). Glia cells in amyotrophic lateral sclerosis: new clues to understanding an old disease? Muscle Nerve 35, 712–724. doi: 10.1002/mus.20768
Ng, S. Y., and Lee, A. Y. W. (2019). Traumatic Brain Injuries: Pathophysiology and Potential Therapeutic Targets. Front. Cell Neurosci. 13:528. doi: 10.3389/fncel.2019.00528
Ngo, V., and Duennwald, M. L. (2022). Nrf2 and Oxidative Stress: A General Overview of Mechanisms and Implications in Human Disease. Antioxidants 11:2345. doi: 10.3390/antiox11122345
Nichols, N. R., Day, J. R., Laping, N. J., Johnson, S. A., and Finch, C. E. (1993). GFAP mRNA increases with age in rat and human brain. Neurobiol. Aging 14, 421–429. doi: 10.1016/0197-4580(93)90100-p
Nielsen, H. M., Ek, D., Avdic, U., Orbjorn, C., Hansson, O., Netherlands Brain, B., et al. (2013). NG2 cells, a new trail for Alzheimer’s disease mechanisms? Acta Neuropathol. Commun. 1:7. doi: 10.1186/2051-5960-1-7
Niu, W., Zang, T., Zou, Y., Fang, S., Smith, D. K., Bachoo, R., et al. (2013). In vivo reprogramming of astrocytes to neuroblasts in the adult brain. Nat. Cell Biol. 15, 1164–1175. doi: 10.1038/ncb2843
Nordengen, K., Kirsebom, B. E., Henjum, K., Selnes, P., Gisladottir, B., Wettergreen, M., et al. (2019). Glial activation and inflammation along the Alzheimer’s disease continuum. J. Neuroinflammation 16:46. doi: 10.1186/s12974-019-1399-2
Norris, C., Weatherbee, J., Murphy, S. F., and VandeVord, P. J. (2023). Quantifying acute changes in neurometabolism following blast-induced traumatic brain injury. Neurosci. Res. doi: 10.1016/j.neures.2023.06.008 [Epub ahead of print].
Nwafor, D. C., Brichacek, A. L., Foster, C. H., Lucke-Wold, B. P., Ali, A., Colantonio, M. A., et al. (2022). Pediatric traumatic brain injury: An update on preclinical models, clinical biomarkers, and the implications of cerebrovascular dysfunction. J. Cent. Nerv. Syst. Dis. 14:11795735221098125. doi: 10.1177/11795735221098125
Obenaus, A., Rodriguez-Grande, B., Lee, J. B., Dubois, C. J., Fournier, M. L., Cador, M., et al. (2023). A single mild juvenile TBI in male mice leads to regional brain tissue abnormalities at 12 months of age that correlate with cognitive impairment at the middle age. Acta Neuropathol. Commun. 11:32. doi: 10.1186/s40478-023-01515-y
O’Brien, W. T., Pham, L., Symons, G. F., Monif, M., Shultz, S. R., and McDonald, S. J. (2020). The NLRP3 inflammasome in traumatic brain injury: potential as a biomarker and therapeutic target. J. Neuroinflammation 17:104. doi: 10.1186/s12974-020-01778-5
Ojha, R. P., Rastogi, M., Devi, B. P., Agrawal, A., and Dubey, G. P. (2012). Neuroprotective effect of curcuminoids against inflammation-mediated dopaminergic neurodegeneration in the MPTP model of Parkinson’s disease. J. Neuroimmune Pharmacol. 7, 609–618. doi: 10.1007/s11481-012-9363-2
Okuma, Y., Liu, K., Wake, H., Zhang, J., Maruo, T., Date, I., et al. (2012). Anti-high mobility group box-1 antibody therapy for traumatic brain injury. Ann. Neurol. 72, 373–384. doi: 10.1002/ana.23602
Ooi, S. Z. Y., Spencer, R. J., Hodgson, M., Mehta, S., Phillips, N. L., Preest, G., et al. (2022). Interleukin-6 as a prognostic biomarker of clinical outcomes after traumatic brain injury: a systematic review. Neurosurg. Rev. 45, 3035–3054. doi: 10.1007/s10143-022-01827-y
Ortega, F. J., Jolkkonen, J., and Rodriguez, M. J. (2013). Microglia is an active player in how glibenclamide improves stroke outcome. J. Cereb. Blood Flow Metab. 33, 1138–1139. doi: 10.1038/jcbfm.2013.72
Osier, N., and Dixon, C. E. (2016). The controlled cortical impact model of experimental brain trauma: overview. Research applications, and protocol. Methods Mol. Biol. 1462, 177–192. doi: 10.1007/978-1-4939-3816-2_11
Otani, N., Nawashiro, H., Fukui, S., Nomura, N., and Shima, K. (2002). Temporal and spatial profile of phosphorylated mitogen-activated protein kinase pathways after lateral fluid percussion injury in the cortex of the rat brain. J. Neurotrauma 19, 1587–1596. doi: 10.1089/089771502762300247
Otani, N., Nawashiro, H., Nagatani, K., Takeuchi, S., Kobayashi, H., and Shima, K. (2011). Mitogen-Activated Protein Kinase Pathways Following Traumatic Brain Injury. Neurosci. Med. 2, 208–216. doi: 10.4236/nm.2011.23028
Palmer, A. M., Marion, D. W., Botscheller, M. L., Swedlow, P. E., Styren, S. D., and DeKosky, S. T. (1993). Traumatic brain injury-induced excitotoxicity assessed in a controlled cortical impact model. J. Neurochem. 61, 2015–2024. doi: 10.1111/j.1471-4159.1993.tb07437.x
Paolicelli, R. C., Sierra, A., Stevens, B., Tremblay, M. E., Aguzzi, A., Ajami, B., et al. (2022). Microglia states and nomenclature: A field at its crossroads. Neuron 110, 3458–3483. doi: 10.1016/j.neuron.2022.10.020
Paredes, I., Navarro, B., and Lagares, A. (2021). Sleep disorders in traumatic brain injury. Neurocirugia 32, 178–187. doi: 10.1016/j.neucie.2020.12.001
Park, E., McKnight, S., Ai, J., and Baker, A. J. (2006). Purkinje cell vulnerability to mild and severe forebrain head trauma. J. Neuropathol. Exp. Neurol. 65, 226–234. doi: 10.1097/01.jnen.0000202888.29705.93
Pang, Z. P., Yang, N., Vierbuchen, T., Ostermeier, A., Fuentes, D. R., Yang, T. Q., et al. (2011). Induction of human neuronal cells by defined transcription factors. Nature 476, 220–223. doi: 10.1038/nature10202
Paudel, Y. N., Angelopoulou, E., Piperi, C., Othman, I., and Shaikh, M. F. (2020). HMGB1-Mediated Neuroinflammatory Responses in Brain Injuries: Potential Mechanisms and Therapeutic Opportunities. Int. J. Mol. Sci. 21, 4609. doi: 10.3390/ijms21134609
Paudel, Y. N., Shaikh, M. F., Chakraborti, A., Kumari, Y., Aledo-Serrano, A., Aleksovska, K., et al. (2018). HMGB1: A common biomarker and potential target for TBI, neuroinflammation, epilepsy, and cognitive dysfunction. Front. Neurosci. 12:628. doi: 10.3389/fnins.2018.00628
Pedersen, T. J., Keil, S. A., Han, W., Wang, M. X., and Iliff, J. J. (2023). The effect of aquaporin-4 mis-localization on Abeta deposition in mice. Neurobiol. Dis. 181:106100. doi: 10.1016/j.nbd.2023.106100
Peeters, W., van den Brande, R., Polinder, S., Brazinova, A., Steyerberg, E. W., Lingsma, H. F., et al. (2015). Epidemiology of traumatic brain injury in Europe. Acta Neurochir. 157, 1683–1696. doi: 10.1007/s00701-015-2512-7
Pekny, M., and Pekna, M. (2016). Reactive gliosis in the pathogenesis of CNS diseases. Biochim. Biophys. Acta 1862, 483–491. doi: 10.1016/j.bbadis.2015.11.014
Penkowa, M., Giralt, M., Lago, N., Camats, J., Carrasco, J., Hernandez, J., et al. (2003). Astrocyte-targeted expression of IL-6 protects the CNS against a focal brain injury. Exp. Neurol. 181, 130–148. doi: 10.1016/s0014-4886(02)00051-1
Perry, V. H., Nicoll, J. A., and Holmes, C. (2010). Microglia in neurodegenerative disease. Nat. Rev. Neurol. 6, 193–201. doi: 10.1038/nrneurol.2010.17
Philips, T., and Rothstein, J. D. (2014). Glial cells in amyotrophic lateral sclerosis. Exp. Neurol. 262, 111–120. doi: 10.1016/j.expneurol.2014.05.015
Pierce, J. E., Smith, D. H., Trojanowski, J. Q., and McIntosh, T. K. (1998). Enduring cognitive, neurobehavioral and histopathological changes persist for up to one year following severe experimental brain injury in rats. Neuroscience 87, 359–369. doi: 10.1016/s0306-4522(98)00142-0
Pinchi, E., Frati, P., Arcangeli, M., Volonnino, G., Tomassi, R., Santoro, P., et al. (2020). MicroRNAs: The New Challenge for Traumatic Brain Injury Diagnosis. Curr. Neuropharmacol. 18, 319–331.
Piwecka, M., Rajewsky, N., and Rybak-Wolf, A. (2023). Single-cell and spatial transcriptomics: deciphering brain complexity in health and disease. Nat. Rev. Neurol. 19, 346–362. doi: 10.1038/s41582-023-00809-y
Plantman, S., Ng, K. C., Lu, J., Davidsson, J., and Risling, M. (2012). Characterization of a novel rat model of penetrating traumatic brain injury. J. Neurotrauma 29, 1219–1232. doi: 10.1089/neu.2011.2182
Plassman, B. L., Havlik, R. J., Steffens, D. C., Helms, M. J., Newman, T. N., Drosdick, D., et al. (2000). Documented head injury in early adulthood and risk of Alzheimer’s disease and other dementias. Neurology 55, 1158–1166. doi: 10.1212/wnl.55.8.1158
Pluta, R., Furmaga-Jablonska, W., Januszewski, S., and Czuczwar, S. J. (2022). Post-ischemic brain neurodegeneration in the form of Alzheimer’s disease proteinopathy: possible therapeutic role of curcumin. Nutrients 14:248. doi: 10.3390/nu14020248
Popkirov, S., Carson, A. J., and Stone, J. (2018). Scared or scarred: Could ‘dissociogenic’ lesions predispose to non-epileptic seizures after head trauma? Seizure 58, 127–132. doi: 10.1016/j.seizure.2018.04.009
Poprac, P., Jomova, K., Simunkova, M., Kollar, V., Rhodes, C. J., and Valko, M. (2017). Targeting Free Radicals in Oxidative Stress-Related Human Diseases. Trends Pharmacol. Sci. 38, 592–607. doi: 10.1016/j.tips.2017.04.005
Porchet, R., Probst, A., Bouras, C., Draberova, E., Draber, P., and Riederer, B. M. (2003). Analysis of glial acidic fibrillary protein in the human entorhinal cortex during aging and in Alzheimer’s disease. Proteomics 3, 1476–1485. doi: 10.1002/pmic.200300456
Porebska, N., Pozniak, M., Matynia, A., Zukowska, D., Zakrzewska, M., Otlewski, J., et al. (2021). Galectins as modulators of receptor tyrosine kinases signaling in health and disease. Cytokine Growth Factor Rev. 60, 89–106. doi: 10.1016/j.cytogfr.2021.03.004
Potolicchio, I., Carven, G. J., Xu, X., Stipp, C., Riese, R. J., Stern, L. J., et al. (2005). Proteomic analysis of microglia-derived exosomes: metabolic role of the aminopeptidase CD13 in neuropeptide catabolism. J. Immunol. 175, 2237–2243. doi: 10.4049/jimmunol.175.4.2237
Pozojevic, J., and Spielmann, M. (2023). Single-Cell Sequencing in Neurodegenerative Disorders. Mol. Diagn. Ther. 27, 553–561. doi: 10.1007/s40291-023-00668-9
Qi, L., Jacob, A., Wang, P., and Wu, R. (2010). Peroxisome proliferator activated receptor-gamma and traumatic brain injury. Int. J. Clin. Exp. Med. 3, 283–292.
Qian, X., Song, H., and Ming, G. L. (2019). Brain organoids: advances, applications and challenges. Development 146, dev166074. doi: 10.1242/dev.166074
Qin, Q., Teng, Z., Liu, C., Li, Q., Yin, Y., and Tang, Y. (2021). TREM2, microglia, and Alzheimer’s disease. Mech. Ageing Dev. 195:111438. doi: 10.1016/j.mad.2021.111438
Qiu, X., Guo, Y., Liu, M. F., Zhang, B., Li, J., Wei, J. F., et al. (2023). Single-cell RNA-sequencing analysis reveals enhanced non-canonical neurotrophic factor signaling in the subacute phase of traumatic brain injury. CNS Neurosci. Ther. 29, 3446–3459. doi: 10.1111/cns.14278
Qu, Z., Zheng, N., Wei, Y., Chen, Y., Zhang, Y., Zhang, M., et al. (2019). Effect of cornel iridoid glycoside on microglia activation through suppression of the JAK/STAT signalling pathway. J. Neuroimmunol. 330, 96–107. doi: 10.1016/j.jneuroim.2019.01.014
Ralay Ranaivo, H., and Wainwright, M. S. (2010). Albumin activates astrocytes and microglia through mitogen-activated protein kinase pathways. Brain Res. 1313, 222–231. doi: 10.1016/j.brainres.2009.11.063
Ramos-Cejudo, J., Wisniewski, T., Marmar, C., Zetterberg, H., Blennow, K., de Leon, M. J., et al. (2018). Traumatic Brain Injury and Alzheimer’s Disease: The Cerebrovascular Link. EBioMedicine 28, 21–30. doi: 10.1016/j.ebiom.2018.01.021
Rane, S. G., and Reddy, E. P. (2000). Janus kinases: components of multiple signaling pathways. Oncogene 19, 5662–5679. doi: 10.1038/sj.onc.1203925
Rao, V. L., Baskaya, M. K., Dogan, A., Rothstein, J. D., and Dempsey, R. J. (1998). Traumatic brain injury down-regulates glial glutamate transporter (GLT-1 and GLAST) proteins in rat brain. J. Neurochem. 70, 2020–2027. doi: 10.1046/j.1471-4159.1998.70052020.x
Redell, J. B., Liu, Y., and Dash, P. K. (2009). Traumatic brain injury alters expression of hippocampal microRNAs: potential regulators of multiple pathophysiological processes. J. Neurosci. Res. 87, 1435–1448. doi: 10.1002/jnr.21945
Reiffurth, C., Berndt, N., Gonzalez Lopez, A., Schoknecht, K., Kovacs, R., Maechler, M., et al. (2023). Deep Isoflurane Anesthesia Is Associated with Alterations in Ion Homeostasis and Specific Na + /K + -ATPase Impairment in the Rat Brain. Anesthesiology 138, 611–623. doi: 10.1097/ALN.0000000000004553
Reiter, R. J., Rosales-Corral, S., Tan, D. X., Jou, M. J., Galano, A., and Xu, B. (2017). Melatonin as a mitochondria-targeted antioxidant: one of evolution’s best ideas. Cell Mol. Life Sci. 74, 3863–3881. doi: 10.1007/s00018-017-2609-7
Reynaert, N. L., Ckless, K., Korn, S. H., Vos, N., Guala, A. S., Wouters, E. F., et al. (2004). Nitric oxide represses inhibitory kappaB kinase through S-nitrosylation. Proc. Natl. Acad. Sci. U. S. A. 101, 8945–8950. doi: 10.1073/pnas.0400588101
Rice, R. A., Spangenberg, E. E., Yamate-Morgan, H., Lee, R. J., Arora, R. P., Hernandez, M. X., et al. (2015). Elimination of Microglia Improves Functional Outcomes Following Extensive Neuronal Loss in the Hippocampus. J. Neurosci. 35, 9977–9989. doi: 10.1523/JNEUROSCI.0336-15.2015
Ritzel, R. M., Doran, S. J., Glaser, E. P., Meadows, V. E., Faden, A. I., Stoica, B. A., et al. (2019). Old age increases microglial senescence, exacerbates secondary neuroinflammation, and worsens neurological outcomes after acute traumatic brain injury in mice. Neurobiol. Aging 77, 194–206. doi: 10.1016/j.neurobiolaging.2019.02.010
Robinson, C., Apgar, C., and Shapiro, L. A. (2016). Astrocyte Hypertrophy Contributes to Aberrant Neurogenesis after Traumatic Brain Injury. Neural Plast. 2016:1347987. doi: 10.1155/2016/1347987
Rochfort, K. D., and Cummins, P. M. (2015). The blood-brain barrier endothelium: a target for pro-inflammatory cytokines. Biochem. Soc. Trans. 43, 702–706. doi: 10.1042/BST20140319
Rodriguez-Grande, B., Obenaus, A., Ichkova, A., Aussudre, J., Bessy, T., Barse, E., et al. (2018). Gliovascular changes precede white matter damage and long-term disorders in juvenile mild closed head injury. Glia 66, 1663–1677. doi: 10.1002/glia.23336
Romine, J., Gao, X., and Chen, J. (2014). Controlled cortical impact model for traumatic brain injury. J. Vis. Exp. 90, e51781. doi: 10.3791/51781
Roof, R. L., Duvdevani, R., Braswell, L., and Stein, D. G. (1994). Progesterone facilitates cognitive recovery and reduces secondary neuronal loss caused by cortical contusion injury in male rats. Exp. Neurol. 129, 64–69. doi: 10.1006/exnr.1994.1147
Rosenfeld, C. S., and Ferguson, S. A. (2014). Barnes maze testing strategies with small and large rodent models. J. Vis. Exp. 84, e51194. doi: 10.3791/51194
Rosenfeld, J. V., McFarlane, A. C., Bragge, P., Armonda, R. A., Grimes, J. B., and Ling, G. S. (2013). Blast-related traumatic brain injury. Lancet Neurol. 12, 882–893. doi: 10.1016/S1474-4422(13)70161-3
Roux, P. P., and Blenis, J. (2004). ERK and p38 MAPK-activated protein kinases: a family of protein kinases with diverse biological functions. Microbiol. Mol. Biol. Rev. 68, 320–344. doi: 10.1128/MMBR.68.2.320-344.2004
Rubiano, A. M., Carney, N., Chesnut, R., and Puyana, J. C. (2015). Global neurotrauma research challenges and opportunities. Nature 527, S193–S197. doi: 10.1038/nature16035
Rubovitch, V., Ten-Bosch, M., Zohar, O., Harrison, C. R., Tempel-Brami, C., Stein, E., et al. (2011). A mouse model of blast-induced mild traumatic brain injury. Exp. Neurol. 232, 280–289. doi: 10.1016/j.expneurol.2011.09.018
Saadoun, S., Papadopoulos, M. C., Watanabe, H., Yan, D., Manley, G. T., and Verkman, A. S. (2005). Involvement of aquaporin-4 in astroglial cell migration and glial scar formation. J. Cell Sci. 118, 5691–5698. doi: 10.1242/jcs.02680
Sahni, V., Mukhopadhyay, A., Tysseling, V., Hebert, A., Birch, D., McGuire, T. L., et al. (2010). BMPR1a and BMPR1b signaling exert opposing effects on gliosis after spinal cord injury. J. Neurosci. 30, 1839–1855. doi: 10.1523/JNEUROSCI.4459-09.2010
Salminen, A., Ojala, J., Kaarniranta, K., Haapasalo, A., Hiltunen, M., and Soininen, H. (2011). Astrocytes in the aging brain express characteristics of senescence-associated secretory phenotype. Eur. J. Neurosci. 34, 3–11. doi: 10.1111/j.1460-9568.2011.07738.x
Sanchez-Gonzalez, R., Koupourtidou, C., Lepko, T., Zambusi, A., Novoselc, K. T., Durovic, T., et al. (2022). Innate immune pathways promote oligodendrocyte progenitor cell recruitment to the injury site in adult zebrafish brain. Cells 11:520. doi: 10.3390/cells11030520
Sanders, M. J., Dietrich, W. D., and Green, E. J. (1999). Cognitive function following traumatic brain injury: effects of injury severity and recovery period in a parasagittal fluid-percussive injury model. J. Neurotrauma 16, 915–925. doi: 10.1089/neu.1999.16.915
Sandhir, R., Onyszchuk, G., and Berman, N. E. (2008). Exacerbated glial response in the aged mouse hippocampus following controlled cortical impact injury. Exp. Neurol. 213, 372–380. doi: 10.1016/j.expneurol.2008.06.013
Schafer, M. J., White, T. A., Iijima, K., Haak, A. J., Ligresti, G., Atkinson, E. J., et al. (2017). Cellular senescence mediates fibrotic pulmonary disease. Nat. Commun. 8:14532. doi: 10.1038/ncomms14532
Schiweck, J., Murk, K., Ledderose, J., Munster-Wandowski, A., Ornaghi, M., Vida, I., et al. (2021). Drebrin controls scar formation and astrocyte reactivity upon traumatic brain injury by regulating membrane trafficking. Nat. Commun. 12:1490. doi: 10.1038/s41467-021-21662-x
Schlett, J. S., Mettang, M., Skaf, A., Schweizer, P., Errerd, A., Mulugeta, E. A., et al. (2023). NF-kappaB is a critical mediator of post-mitotic senescence in oligodendrocytes and subsequent white matter loss. Mol. Neurodegener. 18:24. doi: 10.1186/s13024-023-00616-5
Schwab, N., Grenier, K., and Hazrati, L. N. (2019). DNA repair deficiency and senescence in concussed professional athletes involved in contact sports. Acta Neuropathol. Commun. 7:182. doi: 10.1186/s40478-019-0822-3
Schwab, N., Ju, Y., and Hazrati, L. N. (2021). Early onset senescence and cognitive impairment in a murine model of repeated mTBI. Acta Neuropathol. Commun. 9:82. doi: 10.1186/s40478-021-01190-x
Schwab, N., Taskina, D., Leung, E., Innes, B. T., Bader, G. D., and Hazrati, L. N. (2022). Neurons and glial cells acquire a senescent signature after repeated mild traumatic brain injury in a sex-dependent manner. Front. Neurosci. 16:1027116. doi: 10.3389/fnins.2022.1027116
Seabury, S. A., Gaudette, E., Goldman, D. P., Markowitz, A. J., Brooks, J., McCrea, M. A., et al. (2018). Assessment of Follow-up Care After Emergency Department Presentation for Mild Traumatic Brain Injury and Concussion: Results From the TRACK-TBI Study. JAMA Netw. Open 1, e180210. doi: 10.1001/jamanetworkopen.2018.0210
Sedarous, M., Keramaris, E., O’Hare, M., Melloni, E., Slack, R. S., Elce, J. S., et al. (2003). Calpains mediate p53 activation and neuronal death evoked by DNA damage. J. Biol. Chem. 278, 26031–26038. doi: 10.1074/jbc.M302833200
Selvakumar, G. P., Ahmed, M. E., Iyer, S. S., Thangavel, R., Kempuraj, D., Raikwar, S. P., et al. (2020). Absence of glia maturation factor protects from axonal injury and motor behavioral impairments after traumatic brain injury. Exp. Neurobiol. 29, 230–248. doi: 10.5607/en20017
Sephton, C. F., Cenik, C., Kucukural, A., Dammer, E. B., Cenik, B., Han, Y., et al. (2011). Identification of neuronal RNA targets of TDP-43-containing ribonucleoprotein complexes. J. Biol. Chem. 286, 1204–1215. doi: 10.1074/jbc.M110.190884
Setoguchi, T., Nakashima, K., Takizawa, T., Yanagisawa, M., Ochiai, W., Okabe, M., et al. (2004). Treatment of spinal cord injury by transplantation of fetal neural precursor cells engineered to express BMP inhibitor. Exp. Neurol. 189, 33–44. doi: 10.1016/j.expneurol.2003.12.007
Shandra, O., Winemiller, A. R., Heithoff, B. P., Munoz-Ballester, C., George, K. K., Benko, M. J., et al. (2019). Repetitive diffuse mild traumatic brain injury causes an atypical astrocyte response and spontaneous recurrent seizures. J. Neurosci. 39, 1944–1963. doi: 10.1523/JNEUROSCI.1067-18.2018
Shapira, Y., Shohami, E., Sidi, A., Soffer, D., Freeman, S., and Cotev, S. (1988). Experimental closed head injury in rats: mechanical, pathophysiologic, and neurologic properties. Crit. Care Med. 16, 258–265. doi: 10.1097/00003246-198803000-00010
Sharma, S., Tiarks, G., Haight, J., and Bassuk, A. G. (2021). Neuropathophysiological Mechanisms and Treatment Strategies for Post-traumatic Epilepsy. Front. Mol. Neurosci. 14:612073. doi: 10.3389/fnmol.2021.612073
Shear, D. A., Williams, A. J., Sharrow, K., Lu, X. C., and Tortella, F. C. (2009). Neuroprotective profile of dextromethorphan in an experimental model of penetrating ballistic-like brain injury. Pharmacol. Biochem. Behav. 94, 56–62. doi: 10.1016/j.pbb.2009.07.006
Shen, Y. F., Yu, W. H., Dong, X. Q., Du, Q., Yang, D. B., Wu, G. Q., et al. (2016). The change of plasma galectin-3 concentrations after traumatic brain injury. Clin. Chim. Acta 456, 75–80. doi: 10.1016/j.cca.2016.02.029
Shields, D. C., Haque, A., and Banik, N. L. (2020). Neuroinflammatory responses of microglia in central nervous system trauma. J. Cereb. Blood Flow Metab. 40, S25–S33.
Shimada, I. S., LeComte, M. D., Granger, J. C., Quinlan, N. J., and Spees, J. L. (2012). Self-renewal and differentiation of reactive astrocyte-derived neural stem/progenitor cells isolated from the cortical peri-infarct area after stroke. J. Neurosci. 32, 7926–7940. doi: 10.1523/JNEUROSCI.4303-11.2012
Shiokawa, R., Otani, N., Kajimoto, R., Igarashi, T., Moro, N., Suma, T., et al. (2022). Glibenclamide attenuates brain edema associated with microglia activation after intracerebral hemorrhage. Neurochirurgie 68, 589–594. doi: 10.1016/j.neuchi.2022.07.009
Shitaka, Y., Tran, H. T., Bennett, R. E., Sanchez, L., Levy, M. A., Dikranian, K., et al. (2011). Repetitive closed-skull traumatic brain injury in mice causes persistent multifocal axonal injury and microglial reactivity. J. Neuropathol. Exp. Neurol. 70, 551–567. doi: 10.1097/NEN.0b013e31821f891f
Shultz, S. R., Bao, F., Weaver, L. C., Cain, D. P., and Brown, A. (2013). Treatment with an anti-CD11d integrin antibody reduces neuroinflammation and improves outcome in a rat model of repeated concussion. J. Neuroinflamm. 10:26. doi: 10.1186/1742-2094-10-26
Shultz, S. R., McDonald, S. J., Corrigan, F., Semple, B. D., Salberg, S., Zamani, A., et al. (2020). Clinical relevance of behavior testing in animal models of traumatic brain injury. J. Neurotrauma 37, 2381–2400. doi: 10.1089/neu.2018.6149
Silver, J., and Miller, J. H. (2004). Regeneration beyond the glial scar. Nat. Rev. Neurosci. 5, 146–156. doi: 10.1038/nrn1326
Sim, F. J., Zhao, C., Penderis, J., and Franklin, R. J. (2002). The age-related decrease in CNS remyelination efficiency is attributable to an impairment of both oligodendrocyte progenitor recruitment and differentiation. J. Neurosci. 22, 2451–2459. doi: 10.1523/JNEUROSCI.22-07-02451.2002
Simard, J. M., Geng, Z., Woo, S. K., Ivanova, S., Tosun, C., Melnichenko, L., et al. (2009). Glibenclamide reduces inflammation, vasogenic edema, and caspase-3 activation after subarachnoid hemorrhage. J. Cereb. Blood Flow Metab. 29, 317–330. doi: 10.1038/jcbfm.2008.120
Simard, J. M., Woo, S. K., Schwartzbauer, G. T., and Gerzanich, V. (2012). Sulfonylurea receptor 1 in central nervous system injury: a focused review. J. Cereb. Blood Flow Metab. 32, 1699–1717. doi: 10.1038/jcbfm.2012.91
Simon, C., Gotz, M., and Dimou, L. (2011). Progenitors in the adult cerebral cortex: cell cycle properties and regulation by physiological stimuli and injury. Glia 59, 869–881. doi: 10.1002/glia.21156
Simon, M., Wang, M. X., Ismail, O., Braun, M., Schindler, A. G., Reemmer, J., et al. (2022). Loss of perivascular aquaporin-4 localization impairs glymphatic exchange and promotes amyloid beta plaque formation in mice. Alzheimers Res. Ther. 14:59. doi: 10.1186/s13195-022-00999-5
Simpson, J. E., Ince, P. G., Higham, C. E., Gelsthorpe, C. H., Fernando, M. S., Matthews, F., et al. (2007). Microglial activation in white matter lesions and non-lesional white matter of ageing brains. Neuropathol. Appl. Neurobiol. 33, 670–683. doi: 10.1111/j.1365-2990.2007.00890.x
Skandsen, T., Kvistad, K. A., Solheim, O., Strand, I. H., Folvik, M., and Vik, A. (2010). Prevalence and impact of diffuse axonal injury in patients with moderate and severe head injury: a cohort study of early magnetic resonance imaging findings and 1-year outcome. J. Neurosurg. 113, 556–563. doi: 10.3171/2009.9.JNS09626
Snapper, D. M., Reginauld, B., Liaudanskaya, V., Fitzpatrick, V., Kim, Y., Georgakoudi, I., et al. (2023). Development of a novel bioengineered 3D brain-like tissue for studying primary blast-induced traumatic brain injury. J. Neurosci. Res. 101, 3–19. doi: 10.1002/jnr.25123
Soares, L. C., Al-Dalahmah, O., Hillis, J., Young, C. C., Asbed, I., Sakaguchi, M., et al. (2021). Novel Galectin-3 roles in neurogenesis, inflammation and neurological diseases. Cells 10:3047. doi: 10.3390/cells10113047
Sofroniew, M. V. (2020). Astrocyte Reactivity: Subtypes, States, and Functions in CNS Innate Immunity. Trends Immunol. 41, 758–770. doi: 10.1016/j.it.2020.07.004
Song, S., Hasan, M. N., Yu, L., Paruchuri, S. S., Bielanin, J. P., Metwally, S., et al. (2022). Microglial-oligodendrocyte interactions in myelination and neurological function recovery after traumatic brain injury. J. Neuroinflammation 19:246. doi: 10.1186/s12974-022-02608-6
Sowers, J. L., Sowers, M. L., Shavkunov, A. S., Hawkins, B. E., Wu, P., DeWitt, D. S., et al. (2021). Traumatic brain injury induces region-specific glutamate metabolism changes as measured by multiple mass spectrometry methods. iScience 24:103108. doi: 10.1016/j.isci.2021.103108
Spanos, G. K., Wilde, E. A., Bigler, E. D., Cleavinger, H. B., Fearing, M. A., Levin, H. S., et al. (2007). cerebellar atrophy after moderate-to-severe pediatric traumatic brain injury. AJNR Am. J. Neuroradiol. 28, 537–542.
Stahel, P. F., Smith, W. R., Bruchis, J., and Rabb, C. H. (2008). Peroxisome proliferator-activated receptors: “key” regulators of neuroinflammation after traumatic brain injury. PPAR Res. 2008:538141. doi: 10.1155/2008/538141
Stokum, J. A., Kwon, M. S., Woo, S. K., Tsymbalyuk, O., Vennekens, R., Gerzanich, V., et al. (2018). SUR1-TRPM4 and AQP4 form a heteromultimeric complex that amplifies ion/water osmotic coupling and drives astrocyte swelling. Glia 66, 108–125. doi: 10.1002/glia.23231
Streit, W. J., Sammons, N. W., Kuhns, A. J., and Sparks, D. L. (2004). Dystrophic microglia in the aging human brain. Glia 45, 208–212. doi: 10.1002/glia.10319
Sucha, P., Hermanova, Z., Chmelova, M., Kirdajova, D., Camacho Garcia, S., Marchetti, V., et al. (2022). The absence of AQP4/TRPV4 complex substantially reduces acute cytotoxic edema following ischemic injury. Front. Cell Neurosci. 16:1054919. doi: 10.3389/fncel.2022.1054919
Sun, G., Miao, Z., Ye, Y., Zhao, P., Fan, L., Bao, Z., et al. (2020). Curcumin alleviates neuroinflammation, enhances hippocampal neurogenesis, and improves spatial memory after traumatic brain injury. Brain Res. Bull. 162, 84–93. doi: 10.1016/j.brainresbull.2020.05.009
Sun, P., Liu, D. Z., Jickling, G. C., Sharp, F. R., and Yin, K. J. (2018). MicroRNA-based therapeutics in central nervous system injuries. J. Cereb. Blood Flow Metab. 38, 1125–1148.
Sun, W., Suzuki, K., Toptunov, D., Stoyanov, S., Yuzaki, M., Khiroug, L., et al. (2019). In vivo two-photon imaging of anesthesia-specific alterations in microglial surveillance and photodamage-directed motility in mouse cortex. Front. Neurosci. 13:421. doi: 10.3389/fnins.2019.00421
Taib, T., Leconte, C., Van Steenwinckel, J., Cho, A. H., Palmier, B., Torsello, E., et al. (2017). Neuroinflammation, myelin and behavior: Temporal patterns following mild traumatic brain injury in mice. PLoS One 12:e0184811. doi: 10.1371/journal.pone.0184811
Tan, A. M., Zhang, W., and Levine, J. M. (2005). NG2: a component of the glial scar that inhibits axon growth. J. Anat. 207, 717–725. doi: 10.1111/j.1469-7580.2005.00452.x
Tan, S. W., Zhao, Y., Li, P., Ning, Y. L., Huang, Z. Z., Yang, N., et al. (2021). HMGB1 mediates cognitive impairment caused by the NLRP3 inflammasome in the late stage of traumatic brain injury. J. Neuroinflammation 18:241. doi: 10.1186/s12974-021-02274-0
Tanaka, T., Kai, S., Matsuyama, T., Adachi, T., Fukuda, K., and Hirota, K. (2013). General anesthetics inhibit LPS-induced IL-1beta expression in glial cells. PLoS One 8:e82930. doi: 10.1371/journal.pone.0082930
Tchkonia, T., Zhu, Y., van Deursen, J., Campisi, J., and Kirkland, J. L. (2013). Cellular senescence and the senescent secretory phenotype: therapeutic opportunities. J. Clin. Invest. 123, 966–972. doi: 10.1172/JCI64098
Terpolilli, N. A., Kim, S. W., Thal, S. C., Kuebler, W. M., and Plesnila, N. (2013). Inhaled nitric oxide reduces secondary brain damage after traumatic brain injury in mice. J. Cereb. Blood Flow Metab 33, 311–318. doi: 10.1038/jcbfm.2012.176
Terpolilli, N. A., Zweckberger, K., Trabold, R., Schilling, L., Schinzel, R., Tegtmeier, F., et al. (2009). The novel nitric oxide synthase inhibitor 4-amino-tetrahydro-L-biopterine prevents brain edema formation and intracranial hypertension following traumatic brain injury in mice. J. Neurotrauma 26, 1963–1975. doi: 10.1089/neu.2008.0853
Theadom, A., Mahon, S., Barker-Collo, S., McPherson, K., Rush, E., Vandal, A. C., et al. (2013). Enzogenol for cognitive functioning in traumatic brain injury: a pilot placebo-controlled RCT. Eur. J. Neurol. 20, 1135–1144. doi: 10.1111/ene.12099
Thompson, A. G., Gray, E., Heman-Ackah, S. M., Mager, I., Talbot, K., Andaloussi, S. E., et al. (2016). Extracellular vesicles in neurodegenerative disease - pathogenesis to biomarkers. Nat. Rev. Neurol. 12, 346–357. doi: 10.1038/nrneurol.2016.68
Tisdall, M. M., Rejdak, K., Kitchen, N. D., Smith, M., and Petzold, A. (2013). The prognostic value of brain extracellular fluid nitric oxide metabolites after traumatic brain injury. Neurocrit. Care 19, 65–68. doi: 10.1007/s12028-011-9633-5
Tjalkens, R. B., Popichak, K. A., and Kirkley, K. A. (2017). Inflammatory Activation of Microglia and Astrocytes in Manganese Neurotoxicity. Adv. Neurobiol. 18, 159–181. doi: 10.1007/978-3-319-60189-2_8
Tominaga, T., Shimada, R., Okada, Y., Kawamata, T., and Kibayashi, K. (2019). Senescence-associated-beta-galactosidase staining following traumatic brain injury in the mouse cerebrum. PLoS One 14:e0213673. doi: 10.1371/journal.pone.0213673
Torper, O., Ottosson, D. R., Pereira, M., Lau, S., Cardoso, T., Grealish, S., et al. (2015). In Vivo Reprogramming of Striatal NG2 Glia into Functional Neurons that Integrate into Local Host Circuitry. Cell Rep. 12, 474–481. doi: 10.1016/j.celrep.2015.06.040
Toshkezi, G., Kyle, M., Longo, S. L., Chin, L. S., and Zhao, L. R. (2018). Brain repair by hematopoietic growth factors in the subacute phase of traumatic brain injury. J. Neurosurg. 129, 1286–1294. doi: 10.3171/2017.7.JNS17878
Tsai, C. P., Hu, C., and Lee, C. T. (2019). Finding diseases associated with amyotrophic lateral sclerosis: a total population-based case-control study. Amyotroph. Lateral Scler. Frontotemporal Degener. 20, 82–89. doi: 10.1080/21678421.2018.1522354
Tucker, L. B., Velosky, A. G., and McCabe, J. T. (2018). Applications of the Morris water maze in translational traumatic brain injury research. Neurosci. Biobehav. Rev. 88, 187–200. doi: 10.1016/j.neubiorev.2018.03.010
Uddin, M. S., and Lim, L. W. (2022). Glial cells in Alzheimer’s disease: From neuropathological changes to therapeutic implications. Ageing Res. Rev. 78:101622. doi: 10.1016/j.arr.2022.101622
Uryu, K., Laurer, H., McIntosh, T., Pratico, D., Martinez, D., Leight, S., et al. (2002). Repetitive mild brain trauma accelerates Abeta deposition, lipid peroxidation, and cognitive impairment in a transgenic mouse model of Alzheimer amyloidosis. J. Neurosci. 22, 446–454. doi: 10.1523/JNEUROSCI.22-02-00446.2002
Valko, M., Leibfritz, D., Moncol, J., Cronin, M. T., Mazur, M., and Telser, J. (2007). Free radicals and antioxidants in normal physiological functions and human disease. Int. J. Biochem. Cell Biol. 39, 44–84. doi: 10.1016/j.biocel.2006.07.001
van Landeghem, F. K., Stover, J. F., Bechmann, I., Bruck, W., Unterberg, A., Buhrer, C., et al. (2001). Early expression of glutamate transporter proteins in ramified microglia after controlled cortical impact injury in the rat. Glia 35, 167–179. doi: 10.1002/glia.1082
van Landeghem, F. K., Weiss, T., Oehmichen, M., and von Deimling, A. (2006). Decreased expression of glutamate transporters in astrocytes after human traumatic brain injury. J. Neurotrauma 23, 1518–1528. doi: 10.1089/neu.2006.23.1518
VanGuilder, H. D., Bixler, G. V., Brucklacher, R. M., Farley, J. A., Yan, H., Warrington, J. P., et al. (2011). Concurrent hippocampal induction of MHC II pathway components and glial activation with advanced aging is not correlated with cognitive impairment. J. Neuroinflammation 8:138. doi: 10.1186/1742-2094-8-138
Venkatesan, C., Chrzaszcz, M., Choi, N., and Wainwright, M. S. (2010). Chronic upregulation of activated microglia immunoreactive for galectin-3/Mac-2 and nerve growth factor following diffuse axonal injury. J. Neuroinflammation 7:32. doi: 10.1186/1742-2094-7-32
Vergara, D., Nigro, A., Romano, A., De Domenico, S., Damato, M., Franck, J., et al. (2019). Distinct Protein Expression Networks are Activated in Microglia Cells after Stimulation with IFN-gamma and IL-4. Cells 8:580. doi: 10.3390/cells8060580
Verkerke, M., Hol, E. M., and Middeldorp, J. (2021). Physiological and Pathological Ageing of Astrocytes in the Human Brain. Neurochem. Res. 46, 2662–2675. doi: 10.1007/s11064-021-03256-7
Villapol, S., Loane, D. J., and Burns, M. P. (2017). Sexual dimorphism in the inflammatory response to traumatic brain injury. Glia 65, 1423–1438. doi: 10.1002/glia.23171
Villapol, S., Yaszemski, A. K., Logan, T. T., Sanchez-Lemus, E., Saavedra, J. M., and Symes, A. J. (2012). Candesartan, an angiotensin II AT(1)-receptor blocker and PPAR-gamma agonist, reduces lesion volume and improves motor and memory function after traumatic brain injury in mice. Neuropsychopharmacology 37, 2817–2829. doi: 10.1038/npp.2012.152
Viney, T. J., Sarkany, B., Ozdemir, A. T., Hartwich, K., Schweimer, J., Bannerman, D., et al. (2022). Spread of pathological human Tau from neurons to oligodendrocytes and loss of high-firing pyramidal neurons in aging mice. Cell Rep. 41, 111646. doi: 10.1016/j.celrep.2022.111646
von Streitberg, A., Jakel, S., Eugenin von Bernhardi, J., Straube, C., Buggenthin, F., Marr, C., et al. (2021). NG2-Glia Transiently Overcome Their Homeostatic Network and Contribute to Wound Closure After Brain Injury. Front. Cell Dev. Biol. 9:662056. doi: 10.3389/fcell.2021.662056
Wada, K., Chatzipanteli, K., Busto, R., and Dietrich, W. D. (1999). Effects of L-NAME and 7-NI on NOS catalytic activity and behavioral outcome after traumatic brain injury in the rat. J. Neurotrauma 16, 203–212. doi: 10.1089/neu.1999.16.203
Wake, H., Moorhouse, A. J., Jinno, S., Kohsaka, S., and Nabekura, J. (2009). Resting microglia directly monitor the functional state of synapses in vivo and determine the fate of ischemic terminals. J. Neurosci. 29, 3974–3980. doi: 10.1523/JNEUROSCI.4363-08.2009
Walker, M. G. (2003). Gene expression versus sequence for predicting function: Glia Maturation Factor gamma is not a glia maturation factor. Genom. Proteomics Bioinform. 1, 52–57. doi: 10.1016/s1672-0229(03)01007-6
Waller, R., Baxter, L., Fillingham, D. J., Coelho, S., Pozo, J. M., Mozumder, M., et al. (2019). Iba-1-/CD68 + microglia are a prominent feature of age-associated deep subcortical white matter lesions. PLoS One 14:e0210888. doi: 10.1371/journal.pone.0210888
Walter, A., Finelli, K., Bai, X., Arnett, P., Bream, T., Seidenberg, P., et al. (2017). Effect of Enzogenol(R) Supplementation on Cognitive. Executive, and Vestibular/Balance Functioning in Chronic Phase of Concussion. Dev. Neuropsychol. 42, 93–103. doi: 10.1080/87565641.2016.1256404
Walter, T. J., Suter, R. K., and Ayad, N. G. (2023). An overview of human single-cell RNA sequencing studies in neurobiological disease. Neurobiol. Dis. 184, 106201. doi: 10.1016/j.nbd.2023.106201
Walz, W. (2000). Controversy surrounding the existence of discrete functional classes of astrocytes in adult gray matter. Glia 31, 95–103.
Walz, W., and Lang, M. K. (1998). Immunocytochemical evidence for a distinct GFAP-negative subpopulation of astrocytes in the adult rat hippocampus. Neurosci. Lett. 257, 127–130. doi: 10.1016/s0304-3940(98)00813-1
Wang, C., Ouyang, S., Zhu, X., Jiang, Y., Lu, Z., and Gong, P. (2023). Myricetin suppresses traumatic brain injury-induced inflammatory response via EGFR/AKT/STAT pathway. Sci. Rep. 13, 22764. doi: 10.1038/s41598-023-50144-x
Wang, J., Lu, Y., Carr, C., Dhandapani, K. M., and Brann, D. W. (2023). Senolytic therapy is neuroprotective and improves functional outcome long-term after traumatic brain injury in mice. Front. Neurosci. 17:1227705. doi: 10.3389/fnins.2023.1227705
Wang, C. F., Zhao, C. C., Liu, W. L., Huang, X. J., Deng, Y. F., Jiang, J. Y., et al. (2020). Depletion of Microglia Attenuates Dendritic Spine Loss and Neuronal Apoptosis in the Acute Stage of Moderate Traumatic Brain Injury in Mice. J. Neurotrauma 37, 43–54. doi: 10.1089/neu.2019.6460
Wang, J., Hou, Y., Zhang, L., Liu, M., Zhao, J., Zhang, Z., et al. (2021). Estrogen Attenuates Traumatic Brain Injury by Inhibiting the Activation of Microglia and Astrocyte-Mediated Neuroinflammatory Responses. Mol. Neurobiol. 58, 1052–1061. doi: 10.1007/s12035-020-02171-2
Wanner, I. B., Anderson, M. A., Song, B., Levine, J., Fernandez, A., Gray-Thompson, Z., et al. (2013). Glial scar borders are formed by newly proliferated, elongated astrocytes that interact to corral inflammatory and fibrotic cells via STAT3-dependent mechanisms after spinal cord injury. J. Neurosci. 33, 12870–12886. doi: 10.1523/JNEUROSCI.2121-13.2013
Washington, P. M., Forcelli, P. A., Wilkins, T., Zapple, D. N., Parsadanian, M., and Burns, M. P. (2012). The effect of injury severity on behavior: a phenotypic study of cognitive and emotional deficits after mild, moderate, and severe controlled cortical impact injury in mice. J. Neurotrauma 29, 2283–2296. doi: 10.1089/neu.2012.2456
Webster, K. M., Sun, M., Crack, P. J., O’Brien, T. J., Shultz, S. R., and Semple, B. D. (2019). Age-dependent release of high-mobility group box protein-1 and cellular neuroinflammation after traumatic brain injury in mice. J. Comp. Neurol. 527, 1102–1117. doi: 10.1002/cne.24589
Wesley, U. V., Vemuganti, R., Ayvaci, E. R., and Dempsey, R. J. (2013). Galectin-3 enhances angiogenic and migratory potential of microglial cells via modulation of integrin linked kinase signaling. Brain Res. 1496, 1–9. doi: 10.1016/j.brainres.2012.12.008
White, D. L., Kunik, M. E., Yu, H., Lin, H. L., Richardson, P. A., Moore, S., et al. (2020). Post-Traumatic Stress Disorder is Associated with further Increased Parkinson’s Disease Risk in Veterans with Traumatic Brain Injury. Ann. Neurol. 88, 33–41. doi: 10.1002/ana.25726
Wicher, G., Wallenquist, U., Lei, Y., Enoksson, M., Li, X., Fuchs, B., et al. (2017). Interleukin-33 Promotes Recruitment of Microglia/Macrophages in Response to Traumatic Brain Injury. J. Neurotrauma 34, 3173–3182. doi: 10.1089/neu.2016.4900
Wilhelmsson, U., Li, L., Pekna, M., Berthold, C. H., Blom, S., Eliasson, C., et al. (2004). Absence of glial fibrillary acidic protein and vimentin prevents hypertrophy of astrocytic processes and improves post-traumatic regeneration. J. Neurosci. 24, 5016–5021. doi: 10.1523/JNEUROSCI.0820-04.2004
Wilk, J. E., Herrell, R. K., Wynn, G. H., Riviere, L. A., and Hoge, C. W. (2012). Mild traumatic brain injury (concussion), posttraumatic stress disorder, and depression in U.S. soldiers involved in combat deployments: association with postdeployment symptoms. Psychosom. Med. 74, 249–257. doi: 10.1097/PSY.0b013e318244c604
Williams, A. J., Hartings, J. A., Lu, X. C., Rolli, M. L., Dave, J. R., and Tortella, F. C. (2005). Characterization of a new rat model of penetrating ballistic brain injury. J. Neurotrauma 22, 313–331. doi: 10.1089/neu.2005.22.313
Williams, A. J., Ling, G. S., and Tortella, F. C. (2006). Severity level and injury track determine outcome following a penetrating ballistic-like brain injury in the rat. Neurosci. Lett. 408, 183–188. doi: 10.1016/j.neulet.2006.08.086
Williams, A. J., Wei, H. H., Dave, J. R., and Tortella, F. C. (2007). Acute and delayed neuroinflammatory response following experimental penetrating ballistic brain injury in the rat. J. Neuroinflammation 4, 17. doi: 10.1186/1742-2094-4-17
Willis, E. F., MacDonald, K. P. A., Nguyen, Q. H., Garrido, A. L., Gillespie, E. R., Harley, S. B. R., et al. (2020). Repopulating Microglia Promote Brain Repair in an IL-6-Dependent Manner. Cell 83, e816. doi: 10.1016/j.cell.2020.02.013
Woo, S. K., Tsymbalyuk, N., Tsymbalyuk, O., Ivanova, S., Gerzanich, V., and Simard, J. M. (2020). SUR1-TRPM4 channels, not K(ATP), mediate brain swelling following cerebral ischemia. Neurosci. Lett. 718:134729. doi: 10.1016/j.neulet.2019.134729
Wu, A. G., Yong, Y. Y., Pan, Y. R., Zhang, L., Wu, J. M., Zhang, Y., et al. (2022). Targeting Nrf2-Mediated Oxidative Stress Response in Traumatic Brain Injury: Therapeutic Perspectives of Phytochemicals. Oxid. Med. Cell Longev. 2022:1015791. doi: 10.1155/2022/1015791
Wu, L., Zhao, H., Weng, H., and Ma, D. (2019). Lasting effects of general anesthetics on the brain in the young and elderly: “mixed picture” of neurotoxicity, neuroprotection and cognitive impairment. J. Anesth. 33, 321–335. doi: 10.1007/s00540-019-02623-7
Wu, Y., Zhang, J., Feng, X., and Jiao, W. (2023). Omega-3 polyunsaturated fatty acids alleviate early brain injury after traumatic brain injury by inhibiting neuroinflammation and necroptosis. Transl. Neurosci. 14:20220277. doi: 10.1515/tnsci-2022-0277
Xin, P., Xu, X., Deng, C., Liu, S., Wang, Y., Zhou, X., et al. (2020). The role of JAK/STAT signaling pathway and its inhibitors in diseases. Int. Immunopharmacol. 80:106210. doi: 10.1016/j.intimp.2020.106210
Xiong, Y., Mahmood, A., and Chopp, M. (2013). Animal models of traumatic brain injury. Nat. Rev. Neurosci. 14, 128–142. doi: 10.1038/nrn3407
Xiong, Y., Mahmood, A., and Chopp, M. (2017). Emerging potential of exosomes for treatment of traumatic brain injury. Neural Regen. Res. 12, 19–22. doi: 10.4103/1673-5374.198966
Xu, B., Yu, D. M., and Liu, F. S. (2014). Effect of siRNA-induced inhibition of IL-6 expression in rat cerebral gliocytes on cerebral edema following traumatic brain injury. Mol. Med. Rep. 10, 1863–1868. doi: 10.3892/mmr.2014.2462
Xu, F., Han, L., Wang, Y., Deng, D., Ding, Y., Zhao, S., et al. (2023). Prolonged anesthesia induces neuroinflammation and complement-mediated microglial synaptic elimination involved in neurocognitive dysfunction and anxiety-like behaviors. BMC Med. 21:7. doi: 10.1186/s12916-022-02705-6
Xu, H., Wang, Z., Li, J., Wu, H., Peng, Y., Fan, L., et al. (2017). The Polarization States of Microglia in TBI: A New Paradigm for Pharmacological Intervention. Neural Plast. 2017:5405104. doi: 10.1155/2017/5405104
Xu, J. (2018). New Insights into GFAP Negative Astrocytes in Calbindin D28k Immunoreactive Astrocytes. Brain Sci. 8:143. doi: 10.3390/brainsci8080143
Xu, L., Nguyen, J. V., Lehar, M., Menon, A., Rha, E., Arena, J., et al. (2016). Repetitive mild traumatic brain injury with impact acceleration in the mouse: Multifocal axonopathy, neuroinflammation, and neurodegeneration in the visual system. Exp. Neurol. 275, 436–449. doi: 10.1016/j.expneurol.2014.11.004
Xu, M., Pirtskhalava, T., Farr, J. N., Weigand, B. M., Palmer, A. K., Weivoda, M. M., et al. (2018). Senolytics improve physical function and increase lifespan in old age. Nat. Med. 24, 1246–1256. doi: 10.1038/s41591-018-0092-9
Yakovlev, A. G., Knoblach, S. M., Fan, L., Fox, G. B., Goodnight, R., and Faden, A. I. (1997). Activation of CPP32-like caspases contributes to neuronal apoptosis and neurological dysfunction after traumatic brain injury. J. Neurosci. 17, 7415–7424. doi: 10.1523/JNEUROSCI.17-19-07415.1997
Yamada, K., and Inagaki, N. (2005). Neuroprotection by KATP channels. J. Mol. Cell Cardiol. 38, 945–949. doi: 10.1016/j.yjmcc.2004.11.020
Yamaki, T., Murakami, N., Iwamoto, Y., Sakakibara, T., Kobori, N., Ueda, S., et al. (1998). Cognitive dysfunction and histological findings in rats with chronic-stage contusion and diffuse axonal injury. Brain Res. Brain Res. Protoc. 3, 100–106. doi: 10.1016/s1385-299x(98)00030-0
Yang, L., Wang, F., Yang, L., Yuan, Y., Chen, Y., Zhang, G., et al. (2018). HMGB1 a-Box Reverses Brain Edema and Deterioration of Neurological Function in a Traumatic Brain Injury Mouse Model. Cell Physiol. Biochem. 46, 2532–2542. doi: 10.1159/000489659
Yang, T., Kong, B., Gu, J. W., Kuang, Y. Q., Cheng, L., Yang, W. T., et al. (2014). Anti-apoptotic and anti-oxidative roles of quercetin after traumatic brain injury. Cell Mol. Neurobiol. 34, 797–804. doi: 10.1007/s10571-014-0070-9
Yang, Y., Gao, L., Fu, J., Zhang, J., Li, Y., Yin, B., et al. (2013). Apparent diffusion coefficient evaluation for secondary changes in the cerebellum of rats after middle cerebral artery occlusion. Neural Regen. Res. 8, 2942–2950. doi: 10.3969/j.issn.1673-5374.2013.31.007
Yang, Z., Suzuki, R., Daniels, S. B., Brunquell, C. B., Sala, C. J., and Nishiyama, A. (2006). NG2 glial cells provide a favorable substrate for growing axons. J. Neurosci. 26, 3829–3839. doi: 10.1523/JNEUROSCI.4247-05.2006
Yao, Z., van Velthoven, C. T. J., Kunst, M., Zhang, M., McMillen, D., Lee, C., et al. (2023). A high-resolution transcriptomic and spatial atlas of cell types in the whole mouse brain. Nature 624, 317–332. doi: 10.1038/s41586-023-06812-z
Yee, G., and Jain, A. (2023). Geriatric Head Injury. StatPearls. Treasure Island, FL: StatPearls Publishing.
Yi, J. H., Pow, D. V., and Hazell, A. S. (2005). Early loss of the glutamate transporter splice-variant GLT-1v in rat cerebral cortex following lateral fluid-percussion injury. Glia 49, 121–133. doi: 10.1002/glia.20099
Yin, G., Du, M., Li, R., Li, K., Huang, X., Duan, D., et al. (2018). Glia maturation factor beta is required for reactive gliosis after traumatic brain injury in zebrafish. Exp. Neurol. 305, 129–138. doi: 10.1016/j.expneurol.2018.04.008
Yip, P. K., Carrillo-Jimenez, A., King, P., Vilalta, A., Nomura, K., Chau, C. C., et al. (2017). Galectin-3 released in response to traumatic brain injury acts as an alarmin orchestrating brain immune response and promoting neurodegeneration. Sci. Rep. 7:41689. doi: 10.1038/srep41689
You, J., Youssef, M. M. M., Santos, J. R., Lee, J., and Park, J. (2023). Microglia and astrocytes in amyotrophic lateral sclerosis: Disease-associated states, pathological roles, and therapeutic potential. Biology 12:1307. doi: 10.3390/biology12101307
Yu, F., Shukla, D. K., Armstrong, R. C., Marion, C. M., Radomski, K. L., Selwyn, R. G., et al. (2017). Repetitive Model of Mild Traumatic Brain Injury Produces Cortical Abnormalities Detectable by Magnetic Resonance Diffusion Imaging, Histopathology, and Behavior. J. Neurotrauma 34, 1364–1381. doi: 10.1089/neu.2016.4569
Zahedi, H., Hosseinzadeh-Attar, M. J., Shadnoush, M., Sahebkar, A., Barkhidarian, B., Sadeghi, O., et al. (2021). Effects of curcuminoids on inflammatory and oxidative stress biomarkers and clinical outcomes in critically ill patients: A randomized double-blind placebo-controlled trial. Phytother. Res. 35, 4605–4615. doi: 10.1002/ptr.7179
Zaheer, A., and Lim, R. (1996). In vitro inhibition of MAP kinase (ERK1/ERK2) activity by phosphorylated glia maturation factor (GMF). Biochemistry 35, 6283–6288. doi: 10.1021/bi960034c
Zaheer, A., and Lim, R. (1998). Overexpression of glia maturation factor (GMF) in PC12 pheochromocytoma cells activates p38 MAP kinase, MAPKAP kinase-2, and tyrosine hydroxylase. Biochem. Biophys. Res. Commun. 250, 278–282. doi: 10.1006/bbrc.1998.9301
Zaheer, A., Yorek, M. A., and Lim, R. (2001). Effects of glia maturation factor overexpression in primary astrocytes on MAP kinase activation, transcription factor activation, and neurotrophin secretion. Neurochem. Res. 26, 1293–1299. doi: 10.1023/a:1014241300179
Zaheer, A., Zaheer, S., Sahu, S. K., Knight, S., Khosravi, H., Mathur, S. N., et al. (2007). A novel role of glia maturation factor: induction of granulocyte-macrophage colony-stimulating factor and pro-inflammatory cytokines. J. Neurochem. 101, 364–376. doi: 10.1111/j.1471-4159.2006.04385.x
Zeppenfeld, D. M., Simon, M., Haswell, J. D., D’Abreo, D., Murchison, C., Quinn, J. F., et al. (2017). Association of perivascular localization of aquaporin-4 with cognition and Alzheimer disease in aging brains. JAMA Neurol. 74, 91–99. doi: 10.1001/jamaneurol.2016.4370
Zhang, C., Kang, J., Zhang, X., Zhang, Y., Huang, N., and Ning, B. (2022a). Spatiotemporal dynamics of the cellular components involved in glial scar formation following spinal cord injury. Biomed. Pharmacother. 153:113500. doi: 10.1016/j.biopha.2022.113500
Zhang, X., Zhang, R., Nisa Awan, M. U., and Bai, J. (2022b). The mechanism and function of glia in Parkinson’s disease. Front. Cell Neurosci. 16:903469. doi: 10.3389/fncel.2022.903469
Zhang, L., Wang, H., Zhou, Y., Zhu, Y., and Fei, M. (2018). Fisetin alleviates oxidative stress after traumatic brain injury via the Nrf2-ARE pathway. Neurochem. Int. 118, 304–313. doi: 10.1016/j.neuint.2018.05.011
Zhang, M., Pan, X., Jung, W., Halpern, A. R., Eichhorn, S. W., Lei, Z., et al. (2023). Molecularly defined and spatially resolved cell atlas of the whole mouse brain. Nature 624, 343–354. doi: 10.1038/s41586-023-06808-9
Zhang, S. Z., Wang, Q. Q., Yang, Q. Q., Gu, H. Y., Yin, Y. Q., Li, Y. D., et al. (2019). NG2 glia regulate brain innate immunity via TGF-beta2/TGFBR2 axis. BMC Med. 17:204. doi: 10.1186/s12916-019-1439-x
Zhang, X., Chen, J., Graham, S. H., Du, L., Kochanek, P. M., Draviam, R., et al. (2002). Intranuclear localization of apoptosis-inducing factor (AIF) and large scale DNA fragmentation after traumatic brain injury in rats and in neuronal cultures exposed to peroxynitrite. J. Neurochem. 82, 181–191. doi: 10.1046/j.1471-4159.2002.00975.x
Zhang, Y., Chopp, M., Liu, X. S., Katakowski, M., Wang, X., Tian, X., et al. (2017a). Exosomes derived from mesenchymal stromal cells promote axonal growth of cortical neurons. Mol. Neurobiol. 54, 2659–2673. doi: 10.1007/s12035-016-9851-0
Zhang, Y., Chopp, M., Zhang, Z. G., Katakowski, M., Xin, H., Qu, C., et al. (2017b). Systemic administration of cell-free exosomes generated by human bone marrow derived mesenchymal stem cells cultured under 2D and 3D conditions improves functional recovery in rats after traumatic brain injury. Neurochem. Int. 111, 69–81. doi: 10.1016/j.neuint.2016.08.003
Zhang, Y., Chopp, M., Meng, Y., Katakowski, M., Xin, H., Mahmood, A., et al. (2015). Effect of exosomes derived from multipluripotent mesenchymal stromal cells on functional recovery and neurovascular plasticity in rats after traumatic brain injury. J. Neurosurg. 122, 856–867. doi: 10.3171/2014.11.JNS14770
Zhang, Y. P., Cai, J., Shields, L. B., Liu, N., Xu, X. M., and Shields, C. B. (2014). Traumatic brain injury using mouse models. Transl. Stroke Res. 5, 454–471. doi: 10.1007/s12975-014-0327-0
Zhao, J., Wang, B., Huang, T., Guo, X., Yang, Z., Song, J., et al. (2019). Glial response in early stages of traumatic brain injury. Neurosci. Lett. 708:134335. doi: 10.1016/j.neulet.2019.134335
Zhao, J. B., Zhang, Y., Li, G. Z., Su, X. F., and Hang, C. H. (2011). Activation of JAK2/STAT pathway in cerebral cortex after experimental traumatic brain injury of rats. Neurosci. Lett. 498, 147–152. doi: 10.1016/j.neulet.2011.05.001
Zhao, Z., Loane, D. J., Murray, M. G. II, Stoica, B. A., and Faden, A. I. (2012). Comparing the predictive value of multiple cognitive, affective, and motor tasks after rodent traumatic brain injury. J. Neurotrauma 29, 2475–2489. doi: 10.1089/neu.2012.2511
Zhao, Z. A., Li, P., Ye, S. Y., Ning, Y. L., Wang, H., Peng, Y., et al. (2017). Perivascular AQP4 dysregulation in the hippocampal CA1 area after traumatic brain injury is alleviated by adenosine A(2A) receptor inactivation. Sci. Rep. 7:2254. doi: 10.1038/s41598-017-02505-6
Zheng, F., Zhou, Y. T., Feng, D. D., Li, P. F., Tang, T., Luo, J. K., et al. (2020). Metabolomics analysis of the hippocampus in a rat model of traumatic brain injury during the acute phase. Brain Behav. 10, e01520. doi: 10.1002/brb3.1520
Zhou, Z. L., Xie, H., Tian, X. B., Xu, H. L., Li, W., Yao, S., et al. (2023). Microglial depletion impairs glial scar formation and aggravates inflammation partly by inhibiting STAT3 phosphorylation in astrocytes after spinal cord injury. Neural Regen. Res. 18, 1325–1331. doi: 10.4103/1673-5374.357912
Zhu, X., Bergles, D. E., and Nishiyama, A. (2008). NG2 cells generate both oligodendrocytes and gray matter astrocytes. Development 135, 145–157. doi: 10.1242/dev.004895
Zhu, Y., Doornebal, E. J., Pirtskhalava, T., Giorgadze, N., Wentworth, M., Fuhrmann-Stroissnigg, H., et al. (2017). New agents that target senescent cells: the flavone, fisetin, and the BCL-X(L) inhibitors, A1331852 and A1155463. Aging 9, 955–963. doi: 10.18632/aging.101202
Ziebell, J. M., and Morganti-Kossmann, M. C. (2010). Involvement of pro- and anti-inflammatory cytokines and chemokines in the pathophysiology of traumatic brain injury. Neurotherapeutics 7, 22–30. doi: 10.1016/j.nurt.2009.10.016
Ziebell, J. M., Taylor, S. E., Cao, T., Harrison, J. L., and Lifshitz, J. (2012). Rod microglia: elongation, alignment, and coupling to form trains across the somatosensory cortex after experimental diffuse brain injury. J. Neuroinflammation 9:247. doi: 10.1186/1742-2094-9-247
Keywords: traumatic brain injury, glia, experimental models, neuroinflammation, intercellular signaling, neurodegeneration
Citation: Amlerova Z, Chmelova M, Anderova M and Vargova L (2024) Reactive gliosis in traumatic brain injury: a comprehensive review. Front. Cell. Neurosci. 18:1335849. doi: 10.3389/fncel.2024.1335849
Received: 09 November 2023; Accepted: 01 February 2024;
Published: 28 February 2024.
Edited by:
Maria Grazia Mola, University of Bari Aldo Moro, ItalyReviewed by:
Sudhanshu P. Raikwar, Barrow Neurological Institute (BNI), United StatesIrena Lavrnja, University of Belgrade, Serbia
Copyright © 2024 Amlerova, Chmelova, Anderova and Vargova. This is an open-access article distributed under the terms of the Creative Commons Attribution License (CC BY). The use, distribution or reproduction in other forums is permitted, provided the original author(s) and the copyright owner(s) are credited and that the original publication in this journal is cited, in accordance with accepted academic practice. No use, distribution or reproduction is permitted which does not comply with these terms.
*Correspondence: Lydia Vargova, lydie.vargova@lfmotol.cuni.cz