- 1Centre National de la Recherche Scientifique (CNRS), University of Bordeaux, Laboratoire de Biogenèse Membranaire UMR5200, Villenave d’Ornon, France
- 2Université Paris-Saclay, Institut national de recherche pour l'agriculture, l'alimentation et l'environnement (INRAE), AgroParisTech, Institut Jean-Pierre Bourgin (IJPB), Versailles, France
Since decades plant lipid droplets (LDs) are described as storage organelles accumulated in seeds to provide energy for seedling growth after germination. Indeed, LDs are the site of accumulation for neutral lipids, predominantly triacylglycerols (TAGs), one of the most energy-dense molecules, and sterol esters. Such organelles are present in the whole plant kingdom, from microalgae to perennial trees, and can probably be found in all plant tissues. Several studies over the past decade have revealed that LDs are not merely simple energy storage compartments, but also dynamic structures involved in diverse cellular processes like membrane remodeling, regulation of energy homeostasis and stress responses. In this review, we aim to highlight the functions of LDs in plant development and response to environmental changes. In particular, we tackle the fate and roles of LDs during the plant post-stress recovery phase.
Introduction
Lipids, and in particular triacylglycerols (TAGs), are the most energetic molecules in cells. Yet their hydrophobic nature requires their storage in a specific intracellular structure, the lipid droplets (LDs). Discovered in the 1880s, LDs have only been widely studied since the 2000s, particularly in mammalian cells because of their involvement in several human diseases such as obesity and atherosclerosis (Coleman, 2020). LDs are composed of a central core of neutral lipids, mainly TAGs and sterol esters, surrounded by a monolayer of polar lipids embedding various proteins. Long considered to be inert fat globules, they are now seen as mobile organelles, found in most of organisms from archaea to eukaryotes (Lundquist et al., 2020).
In plants, two compartments are dedicated to the storage of neutral lipids: lipid droplets in the cytosol, also known as oleosomes, spherosomes, or oil bodies, and plastoglobules within plastids (Bréhélin et al., 2007). Cytosolic LDs have been intensively studied in seeds because of their economic importance. However, it is now known that they are also present in pollen and diverse vegetative organs (Figure 1). Plastoglobules are thylakoid membrane-bound compartments with a structure similar to LDs. In addition to neutral lipids, they also contain secondary metabolites as carotenoids, plastoquinones, and vitamins (tocopherol and phylloquinone). They are involved in various physiological processes such as lipid metabolism and stress responses (reviewed by van Wijk and Kessler, 2017). The proteome of plastoglobules revealed the presence of FIBRILLINS, a family of structural proteins absent from LDs (Lundquist et al., 2012). The functions of FIBRILLINS and plastoglobules have been recently extensively described (Michel et al., 2021; Arzac et al., 2022; Kim and Kim, 2022) and will not be addressed in this review.
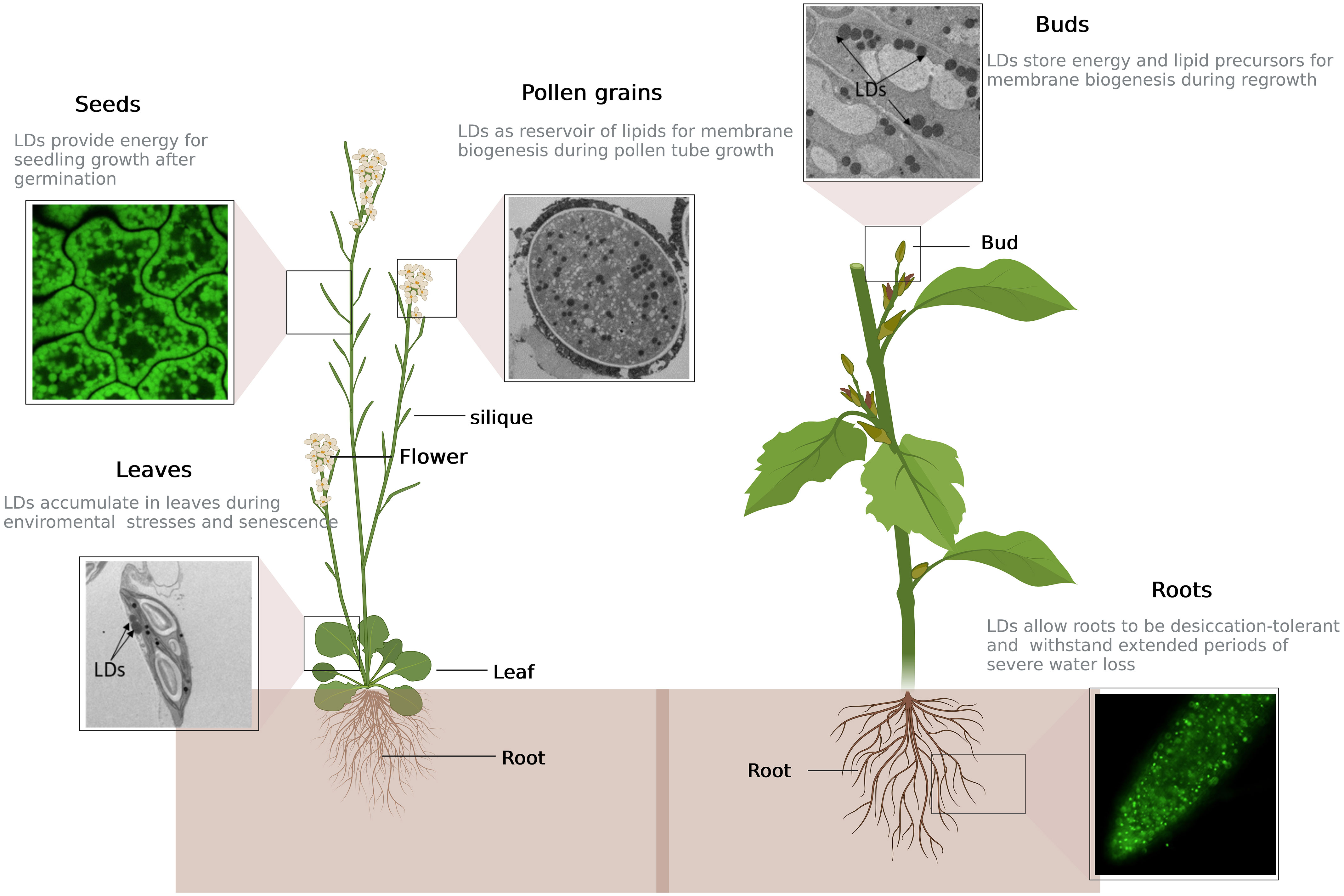
Figure 1 Repartition and function of lipid droplets in different plant tissues. Depending on developmental stage and physiological state of the plant, lipid droplets (LDs) can be present either in seeds or vegetative tissues such as roots, leaves, dormant buds and pollen grains. Top left: cell of Arabidopsis seed stained with BODIPY, a LD-specific dye, and observed by confocal microcopy; center: Arabidopsis pollen grain filled with LDs (in black), observed by electron microscopy; top right: picture of dormant sweet cherry (Prunus avium) floral bud by electron microscopy; bottom left: cell of Arabidopsis leaf after five days of nitrogen starvation showing two LDs in contact with chloroplast, observed by electron microscopy; bottom right: LDs in Arabidopsis root tip labelled in green by overexpression of LDAP1-YFP, after two days of nitrogen starvation, observed by confocal microscopy. This figure was created at BioRender.com.
In recent decades, the mechanistic details of plant LD biogenesis have become clearer (for review see Chapman et al., 2019; Choi et al., 2022; Guzha et al., 2023). Briefly, LD biosynthesis occurs in a three-step process: (i) TAG synthesis at the ER, (ii) TAG accumulation between the two leaflets of the endoplasmic reticulum (ER) membrane leading to the formation of a lens-like structure, and (iii) Budding of the lipid droplet from the ER leaflet into the cytosol. Two pathways permit the synthesis of TAGs: the first one is an acyl-Coenzyme A (acyl-CoA) dependent pathway, called the Kennedy pathway, in which glycerol-3-phosphate is converted to phosphatidic acid (PA) by the sequential esterification of two acyl-CoA to the glycerol 3-phosphate catalysed by two acyltransferases: the GLYCEROL-3-PHOSPHASTE ACYLTRANSFERASE (GPAT) and the LYSO-PHOSPHATIDIC ACID ACYLTRANSFERASE (LPAT), and thereafter PA is dephosphorylated by the PHOSPHATIDIC ACID PHOSPHATASE (PAP) to give diacylglycerol (DAG). A finale acylation of DAG to TAG then occurs, catalysed by the DIACYLGLYCEROL ACYLTRANSFERASE (DGAT). The second pathway involves the PHOSPHOLIPID : DIACYLGLYCEROL ACYLTRANSFERASE (PDAT), which does not use acyl-CoA as acyl donor but transfers an acyl group from the phospholipid phosphatidylcholine, to DAG (Figure 2; for more details, see Xu and Shanklin, 2016). Neutral lipid accumulation occurs at specific location in the ER, where LDAP-INTERACTING PROTEIN (LDIP) interacts with SEIPIN 2/3 proteins (Coulon et al., 2020; Pyc et al., 2021). During LD budding, LDIP dissociates from SEIPIN complex and interacts with a LD surface protein, LIPID DROPLET ASSOCIATED PROTEIN (LDAP). At the same time, VESICLE-ASSOCIATED MEMBRANE PROTEIN (VAMP) -ASSOCIATED PROTEIN 27-1 (VAP27-1), an ER protein, interacts with SEIPIN2 and/or SEIPIN3 to stabilize the LD-forming complex (Greer et al., 2020). These contact sites between LD and ER membrane have been evidenced by tomography in Arabidopsis leaves (Brocard et al., 2017). It is not clearly determined whether plant LDs may, in a final step, detach from the ER or remain attached to it. The mechanisms that would trigger and control the cleavage of LDs from the ER are totally unknown.
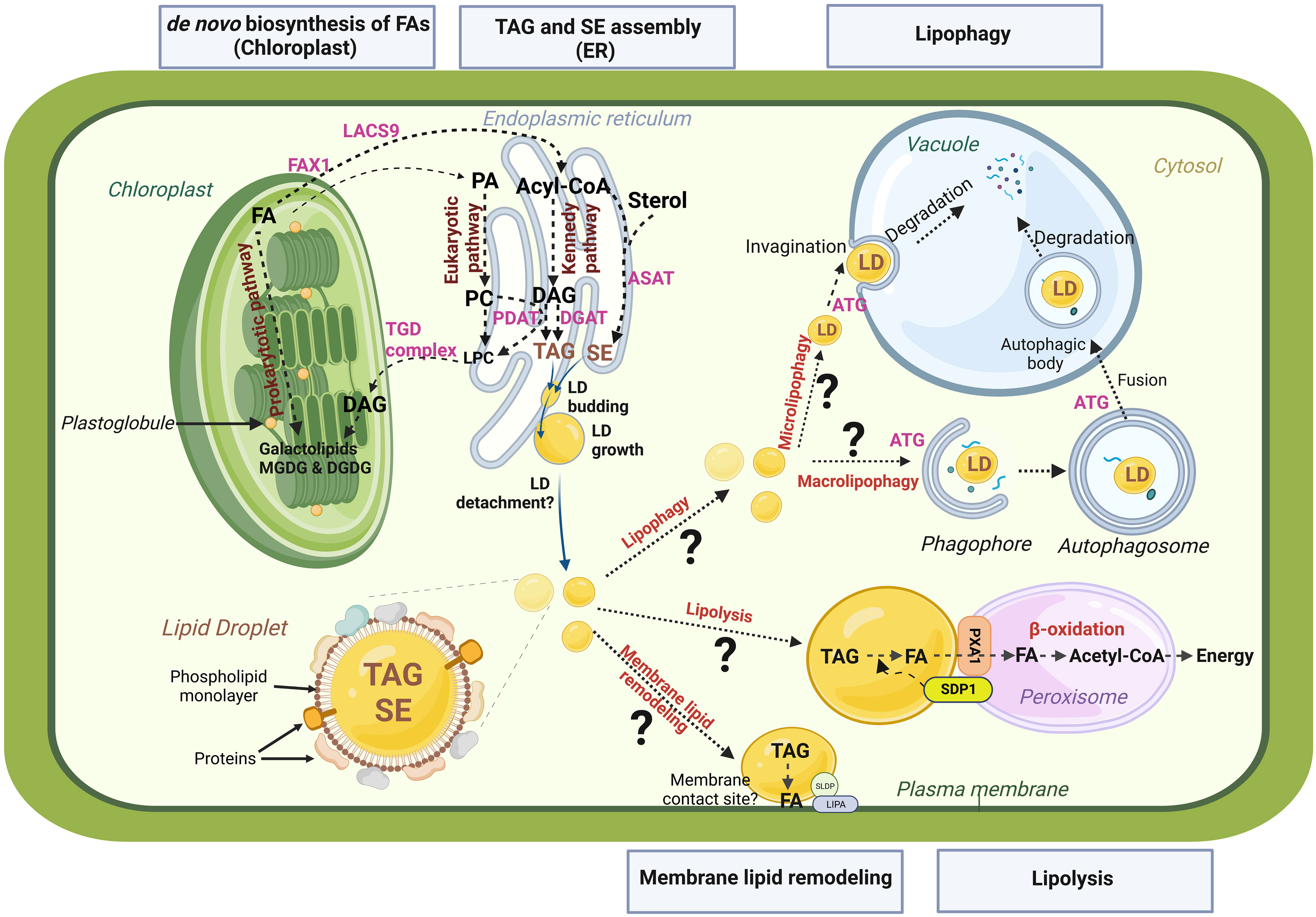
Figure 2 Schematic representation of LD life cycle and potential remobilization pathways. Biosynthesis of fatty acids (FAs) takes place in the chloroplast. Biosynthesis of plastidial galactolipids (MGDG, monogalactosyldiacylglycerol and DGDG, digalactosyldiacylglycerol) occuring within the chloroplast is termed the chloroplastic or ‘‘prokaryotic “ pathway, and the one in the endoplasmic reticulum (ER), that involves phospholipid synthesis (PA, phosphatidic acid; PC, phosphatidylcholine) and subsequent transfer to the chloroplast constitutes the endoplasmic or “ eukaryotic “ pathway. When exported to the cytosol, free FAs are first transported by FA EXPORT 1 protein (FAX1) accross the inner membrane and then converted to activated acyl-CoA by the LONG ACYL-COA SYNTHETASE 9 (LACS9). Acyl-coA are transported to the ER for TAG (triacylglycerol) and SE (sterol ester) assemblies. The major pathway for TAG synthesis is the Kennedy pathway. Diacylglycerol (DAG) is the direct precursor for TAG synthesis. DAG can be converted to TAG by DGAT (DIACYLGLYCEROL ACYLTRANSFERASE) or PDAT (PHOSPHOLIPID DIACYGLYCEROL ACYLTRANSFERASE), using acyl-CoA or PC as acyl donor, respectively. SEs are synthetized from free sterols by the enzymes PSAT (PHOSPHOLIPID STEROL ACYLTRANSFERASE) or ASAT (ACYL-COA STEROL ACYLTRANSFERASE). TAGs and SEs accumulate between the two leaflets of the ER, leading to the formation of a lens-like structure and the budding of a new lipid droplet (LD). Lipolysis and lipophagy are the two main pathways for LD remobilization. Lipolysis involves lipases like SDP1 (SUGAR DEPENDENT PROTEIN 1). PXA1 is a peroxisomal transporter required for uptake of FAs from LD to peroxisome for β-oxidation. Two distinct pathways of lipophagy may be at play: microlipophagy and macrolipophagy. Microphagy involves the invagination of tonoplast to trap LDs within the vacuole. Macrophagy involves the formation of double membrane vesicles called autophagosomes that will sequester LDs and bring them to vacuole for degradation. Both microlipophagy and macrolipophagy involve autophagy related preteins (ATG). TAGs within LDs could also be a source of FAs for lipid membrane remodeling thanks to enzymes that remain to be identified and through organelle contacts maintained by tethering proteins such as SLDP (SEED LD PROTEIN) and LIPA (LD-PLASMA MEMBRANE ADAPTATOR) at LD-plasma membrane connections. This figure was created at BioRender.com.
Several proteomics studies conducted on plant LDs from diverse tissues and organ origins, such as hybrid aspen buds (Veerabagu et al., 2021), mesocarp and seed tissues of Chinese tallow (Zhi et al., 2017), nutsedge tubers (Niemeyer et al., 2022), tobacco pollen tubes (Kretzschmar et al., 2018), Arabidopsis seedlings (Kretzschmar et al., 2020), drought-stressed (Doner et al., 2021) or senescent Arabidopis leaves (Brocard et al., 2017) have led to the identification of hundreds of LD-associated proteins. Regularly, scientific reviews describe the newly discovered mechanisms that participate to the regulation of plant LD biogenesis, and list the function of each LD-associated protein. LDs in algae, particularly in the oil-rich diatom Phaeodactylum tricornutum or in the model green algae Chlamydomonas reinhardtii, are also in the scientist’s spotlight since the two past decades due to their potential use as a platform for biofuel production (reviewed in Kong et al., 2018). Notably, LD proteomes of different algae species have been determined, showing the presence of an abundant structural protein such as MAJOR LIPID DROPLET PROTEIN (MLDP) in C. reinhardtii and Dunaliella salina (Moellering and Benning, 2010; Nguyen et al., 2011; Davidi et al., 2012) or LIPID DROPLET SURFACE PROTEIN (LDSP) in Nannochloropsis oceanica (Vieler et al., 2012), but also of different proteins, more numerous and varied than in land plant LDs, among which are in particular enzymes of the lipid metabolism (reviewed in Goold et al., 2015). While the role of seed LDs as energy reservoirs for the post-germination phase is well known, the function of LDs in non-seed tissues is still far from being elucidated. The aim of the present review is thus to highlight the physiological functions that LDs may play in land plants, from roots to pollen grains (see Figure 1), and in particular the role they may have in the plant response to environment changes. Therefore, will we focus our description to LDs of land plants, without any reference to algae LDs.
LDs are found in many plant organs, where they play various roles
Seed LDs function in germination and post-germinative growth
Seed LD heterogeneity, a sign of functional diversity?
Neutral lipids, mainly TAGs, accumulate to high levels in the seeds of oilseed crops, but are likely present in all seeds. These storage lipids are packaged in micrometer-sized cytosolic LDs distributed in several different types of seed tissue. The abundance and distribution of LDs between seed tissues vary considerably among plant species (Murphy, 2001). In exalbuminous dicotyledons such as rapeseed, sunflower, soybean, and the model plant Arabidopsis thaliana, LDs are predominantly stored in the embryo axis and cotyledons. However, the endosperm of these oilseeds, although smaller than embryonic tissues, also contains LDs (Penfield et al., 2004; Miray et al., 2021). In contrast, seeds from albuminous dicotyledons like castor bean and coriander, and from monocots like palm oil or coconut accumulate LDs mainly in their large endosperm tissue, whereas embryonic tissues are relatively poor in lipids. The heterogeneity of seed LDs relates not only to their tissue distribution and abundance but also to their composition. Embryonic tissues and endosperm display contrasting oil composition, as shown by fatty acid (FA) analysis of tissues separated by dissection from various oilseeds such as oil palm and Arabidopsis (Li et al., 2006; Dussert et al., 2013; Miray et al., 2021). A heterogeneous oil composition was also observed within the embryonic tissues by in situ mapping of lipid molecular species onto seed sections using matrix-assisted laser/desorption ionization-mass spectrometry imaging (MALDI-MSI). The uneven spatial distribution of TAG species between hypocotyl and cotyledons was indeed demonstrated in various oilseeds (Horn et al., 2012; Sturtevant et al., 2017; Woodfield et al., 2017; Sturtevant et al., 2020). LD heterogeneity is not only related to lipids, but also to proteins associated with the LD surface. While the most abundant proteins of LDs from oilseed species belong to the oleosin (OLE) family (Jolivet et al., 2009; Huang, 2018), two other families of LD-associated proteins, i.e. steroleosins and caleosins, are also characteristic of seed LDs. Oleosins are structural proteins controlling LD size and stability in mature seeds, probably by shielding LD surface and thus preventing LD-LD fusions during seed dessication and rehydration (Siloto et al., 2006; Miquel et al., 2014). On the contrary, caleosins and steroleosins are considered to have enzymatic functions. Steroleosins display hydrosteroid deshydrogenase activity and may play a role in sterol signaling, but their natural substrates remain to be determined (Lin et al., 2002; d’Andréa et al., 2007;Zhang et al., 2016). Caleosins are calcium-binding peroxygenases that associate with seed LDs from many species (Chapman et al., 2012). While the caleosin CLO3 is involved in the production of a phytoalexin in leaves (Shimada et al., 2014, see below), seed-expressed caleosins such as CLO1 modulate LD accumulation and mobilization (Poxleitner et al., 2006; Liu et al., 2022). To date, seed LD proteomes have been deciphered in several species after isolation of LDs from whole seeds (Jolivet et al., 2013; Wang et al., 2016; Kretzschmar et al., 2020). However, when the different seed lipid-rich tissues were separated prior to LD isolation, proteomic analyses revealed tissue specificity in LD protein composition. In jojoba, LDs isolated from the embryonic axis contain a lower amount of oleosins and a higher amount of caleosins and steroleosins than LDs from cotyledons (Sturtevant et al., 2020). In rice, caleosins specifically associate with embryo LDs, in contrast to aleurone layer, which contains only oleosins (Chen et al., 2012). Thus, although the primary function of oilseed LDs is related to energy storage, the coexistence of distinct LD pools in different seed tissues raises the question of whether some LDs with specific distribution and composition may have other functions than providing carbon and energy for seedling establishment. These functions could be related to germinative or post-germinative processes important in both oilseed and non-oilseed species, including provision of lipid building blocks for membrane expansion of organelles such as glyoxysomes (Chapman and Trelease, 1991), oxylipin signaling in response to environmental stress (Hanano et al., 2023), and control of dormancy. This last function, specific to seeds, is supported by a few reports showing that changes in LD structure and composition alter seed dormancy in Arabidopsis. Seed dormancy, an innate state in which viable seeds do not germinate even under favorable environmental conditions, is broken by dry storage or by a cold imbibition. Transgenic lines overexpressing HSD1 steroleosin exhibit lower cold and light requirements to break seed dormancy than wild type (Li et al., 2007; Baud et al., 2009). More recently, Taurino et al. (2018), showed that seipin2 mutant seeds display increased dormancy while the final germination rate is not affected. Seipin2 seeds have enlarged LDs compared to wild-type seeds, due to alteration in LD biogenesis during embryo development, which suggests that LD size may influence the degree of seed dormancy.
LD protein remodeling and LD-peroxisome association are central to LD mobilization in seeds
The molecular mechanisms of seed LD mobilization are best described in Arabidopsis and occur during post-germinative growth. Indeed, while the germination is largely driven by the metabolism of non-lipid storage reserves and endosperm storage lipids (Penfield et al., 2004), seedling growth and development relies on the massive and rapid degradation of TAGs that begins once germination is complete, i.e. when the radicle has emerged (Eastmond et al., 2000; Graham, 2008). The conversion of seed TAGs to sucrose occurs through sequential metabolic steps catalyzed by well-known enzymes (see Ischebeck et al., 2020; Zienkiewicz and Zienkiewicz, 2020; Choi et al., 2022 for reviews). Briefly, SUGAR-DEPENDENT 1 (SDP1) and SDP1-LIKE (SDP1-L) lipases cleave TAGs to produce DAGs and free FAs (Eastmond, 2006; Kelly et al., 2011). FAs are then degraded by β-oxidation in specialized peroxisomes called glyoxysomes, to generate sucrose through the glyoxylate cycle and the gluconeogenesis pathway. Additional steps are required before peroxisomal β-oxidation, including transport into peroxisomes through the PEROXISOMAL ATP-BINDING CASSETTE TRANSPORTER PXA1 (Zolman et al., 2001) and activation of FA to acyl-CoA by the LONG-CHAIN ACYL-COA SYNTHETASES LACS6 and LACS7 (Fulda et al., 2004). Arabidopsis mutants deficient in SDP1/SDP1-L, LACS6/LACS7 or PXA1 are all defective in seed LD mobilization and require exogenous sucrose for seedling establishment, demonstrating that the primary role of seed LDs is to provide sugars and energy for seedling establishment. SDP1 and SDP1-L account for at least 90% of the TAG lipase activity in Arabidopsis seedlings, but are not involved in the subsequent breakdown of DAGs and monoacylglycerols (MAGs) (Kelly et al., 2011). To date, several candidates have been characterized for lipase activity towards TAG, DAG and MAG, but their contribution to seed oil mobilization has been ruled out or not established (Kim R, et al., 2016; Müller and Ischebeck, 2018; Choi et al., 2022).
The metabolic switch from quiescent to dynamic LDs that occurs during seed germination involves intense remodeling of the LD proteome and the establishment of close associations between LDs and glyoxysomes. The major seed LD proteins, i.e. oleosins, caleosins and steroleosins, are progressively degraded and replaced by, among others, LD structural proteins of the LDAP family (Kretzschmar et al., 2020). Remodeling of the LD proteome is most likely a prerequisite for lipolysis, as oleosins are considered to form a protective coat on the surface of LDs, preventing lipases from accessing their TAG substrates prior to seed germination (D’Andrea, 2016). Oleosin degradation during germination depends on their ubiquitination, a post-translational modification also reported for caleosins and steroleosins (Deruyffelaere et al., 2015; Kretzschmar et al., 2020). The addition of a diubiquitin linked on lysine 48 marks oleosins for proteasomal degradation (Deruyffelaere et al., 2015). To be accessible to the cytosolic proteasome for degradation, ubiquitinated oleosins are first dislocated from the LD surface by the CELL DIVISION CYCLE 48A (CDC48A) AAA-type ATPase. Central to this mechanism named LD-Associated Degradation (LDAD) is the adaptor PLANT UBX DOMAIN-CONTAINING PROTEIN 10 (PUX10), which connects CDC48A with ubiquitinated oleosins to promote their extraction from LDs and facilitate their proteasomal degradation (Deruyffelaere et al., 2018; Kretzschmar et al., 2018).
In postgerminative seedlings, LDs are physically and functionally associated with peroxisomes (glyoxysomes). Peroxisomes congregate near LDs to facilitate FA import for glyoxysomal degradation and expansion of glyoxysome membrane (Chapman and Trelease, 1991; Hayashi et al., 2001). Blockage of lipid mobilization in the β-oxidation-deficient peroxisome defective 1 (ped1) mutant or in the lipolysis-deficient sdp1 mutant results in enlarged peroxisomes, which exhibit membrane invagination at the site of interaction with an LD (Hayashi et al., 2001; Cui et al., 2016). In contrast, exogenous sucrose supplementation reduces the physical interaction between LDs and peroxisomes. These observations suggest that cellular sucrose level regulates the LD-peroxisome association, which in turn controls sugar production from storage lipids (Cui et al., 2016). SDP1 lipase is first localized to the peroxisomal membrane and then delivered to LDs by direct contact or via tubular extensions of the peroxisome membrane, called peroxules (Thazar-Poulot et al., 2015). The retromer trafficking machinery is involved in the formation of peroxules through a mechanism that remains to be identified (Thazar-Poulot et al., 2015). Recently, Huang et al. (2022) reported that FYVE DOMAIN PROTEIN REQUIRED FOR ENDOSOMAL SORTING 1 (FREE1), a component of the endosomal sorting complex for transport (ESCRT), regulates peroxisomal tubulation and SDP1 targeting to LDs. Consistently, free1 loss-of-function mutant is impaired in LD degradation in postgerminative seedlings. The mechanism is not yet understood, but involves the peroxin PEX11e, a peroxisomal protein that plays key roles in peroxisomal proliferation and tubulation, and SDP1, both of which interact with FREE1.
Autophagy is probably also involved in LD mobilization
Apart from lipolysis, LDs can be degraded by lipophagy, which refers to the autophagic degradation of LDs in the vacuole. In plants, lipophagy is poorly described and reported in pollen, and in dark-stressed plantlets (Kurusu et al., 2014; Fan et al., 2019a). Several lines of evidence suggest that lipophagy could also be active during early seedling growth. In Arabidopsis, Avin-Wittenberg et al. (2015) demonstrated that autophagy-deficient atg5 (autophagy related gene 5) and atg7 seedlings have shorter hypocotyls than wild type when grown in darkness. This phenotype was reversed by providing exogenous sucrose, indicating that autophagy contributes to carbon supply, likely by degrading LDs, in young seedlings. The presence of LDs in the vacuole has been observed by transmission electron microscopy in Arabidopsis and castor bean seedlings (Poxleitner et al., 2006; Han et al., 2020), suggesting that LDs could be degraded in the vacuole. Moreover, ATG8 proteins, which are major players of the autophagy pathway, were shown to interact with seed LD proteins. Marshall et al. (2019) demonstrated that AtOLE1 binds to AtATG8e through an ATG8 interacting motif (AIM). Very recently, in a not yet peer-reviewed manuscript, AtCLO1 was also shown to contain two AIM domains, one of which seems required for its interaction with ATG8b (Miklaszewska et al., 2023). Interestingly, CLO1 deficiency was consistently shown to delay LD mobilization and to prevent the transfer of LDs to the vacuole in post-germinative seedlings. The translocation of LDs into the vacuoles was shown to occur by invagination of the tonoplast, in a process that resembles microautophagy (Poxleitner et al., 2006). Overall, these observations support the hypothesis of an autophagy-dependent vacuolar degradation of seed LDs, but the physiological role and regulatory mechanisms of this alternative pathway to lipolysis remain unknown.
LDs accumulation in pollen grains is crucial for pollen fertility
Numerous ultrastructural studies have documented the presence of large amounts of LDs within pollen grains (reviewed in Piffanelli et al., 1998). These LDs accumulate during pollen grain maturation (Rotsch et al., 2017) and have been demonstrated to store mostly TAGs (Piffanelli et al., 1997; Hernández et al., 2020). For example, TAGs represent up to 39% of the intracellular lipids of the mature pollen grain of Brassica napus (Piffanelli et al., 1997). TAG biosynthesis and LD accumulation seems to be critical for pollen development. Indeed, pollen from mutant impaired in TAG biosynthesis, such as the dgat1 pdat1 double mutant is sterile (Zhang et al., 2009). The mutation of both transcription factors wrky2 wrky34 in which genes involved in TAG synthesis are repressed and LD abundancy is reduced in pollen grains (Zheng et al., 2018), also results in pollen sterility (Guan et al., 2014). During pollen grain germination, LDs exit from the grain and accumulate in the growing tube (Rodríguez-García et al., 2003; Zienkiewicz et al., 2013). TAG synthesis during pollen tube growth has been demonstrated by feeding germinating pollens with radioactive ethanol and sucrose, but lipase activity has also been detected in pollen grain and tube (Zienkiewicz et al., 2013). The importance of the TAG lipase activity in pollen germination has been evidenced using the LD-located lipase obl1 (oil body lipase 1) mutant in which the pollen germination is hampered (Müller and Ischebeck, 2018). In addition, AtSDP1-L shows exceptionally high expression in mature pollen grains (Kelly et al., 2011), suggesting that AtSDP1-L may be involved in LD breakdown during pollen germination. Indeed, FA composition of TAGs constantly varies during pollen germination, suggesting that TAG mobilization and synthesis both happen in the growing pollen tube (Hernández et al., 2020). In contrast to seeds where TAGs are used as a source of energy for the plantlet post-germinative growth, pollen TAGs rather represent a reservoir of lipids to provide FAs necessary for de novo membrane biogenesis during pollen tube growth (Mellema et al., 2002; Ischebeck, 2016; Hernández et al., 2020). The essential role of LDs in pollen fertilization efficiency is exemplified by mutants in LD morphology such as the seipin2 seipin3 double mutant (Taurino et al., 2018) and the pald (protein associated with lipid droplets) one (Li et al., 2022), which are impaired in tube germination and pollen longevity, respectively. It can be speculated that FAs are biosynthesized in the pollen grain where nutrients are available, and stored as TAGs within LDs, which are then transported to tip region of the pollen tube where most membrane components are needed. LD transport may happen on the actin filaments present in the pollen tube (Fu, 2015), similarly to what has already been described in root hairs (Veerabagu et al., 2020). As mobile reservoir of FAs, LDs would provide lipids necessary for membrane synthesis during pollen tube growth but also facilitate acyl editing of the lipid membrane and modulate the membrane fluidity in response for example to temperature changes during tube growth (Ischebeck, 2016; Krawczyk et al., 2022a).
LD abundancy in buds of perennial trees varies during the course of dormancy
Similar to seeds, buds of perennial species establish dormancy, partially desiccate to tolerate frost, and accumulate LDs. Indeed, the presence of LDs in buds has been reported in several perennial species such as birch (Rinne et al., 1999; Rinne et al., 2001), Norway spruce (Guzicka and Wozny, 2003; Guzicka et al., 2018), aspen and poplar (Sagisaka, 1992; Rinne et al., 2011; Veerabagu et al., 2020; Veerabagu et al., 2021), willow (Berggren, 1984), pine (Jordy, 2004) and Cunninghamia lanceolate (Xu et al., 2016). LD abundancy is described to vary during winter dormancy progression (Rinne et al., 2001; Sutinen et al., 2009; Sutinen et al., 2012). LDs already present in growing shoot apex, accumulate in quiescent buds during dormancy and are remobilized during bud development once dormancy is released either naturally, or artificially for example by gibberellin (GA4) treatment (Rinne et al., 2001; Jordy, 2004; Rinne et al., 2011; Sutinen et al., 2012; Veerabagu et al., 2020; Veerabagu et al., 2021). Recently, Veerabagu et al. (2021) determined the proteome of an LD enriched fraction obtained from poplar dormant buds. Four LDAP isoforms were detected in the bud LD enriched fraction, and the expression of the six LDAP genes was highly upregulated in dormant buds compared to developing ones. In addition, LDIP and CLO1 were also present in LD enriched fraction from dormant buds. On the contrary, the expression of three OLE genes was high in developing buds but no oleosin was detected in dormant buds. This suggests that LDAPs replace oleosins in LDs during bud maturation, in contrast to what happens during seed development (Kretzschmar et al., 2020). Whether the LD status may regulate bud dormancy, similarly to what has been described in Arabidopsis seeds (Taurino et al., 2018) remains to be investigated.
In addition to their role as energy storage, LDs in buds have been demonstrated to target plasmodesmata during dormancy release. These LDs deliver callose-hydrolyzing 1,3-β-glucanases to remove callose that was deposited during dormancy set to physically close plasmodesmata (Rinne et al., 2001; Rinne et al., 2011). The directional trafficking of LDs to plasmodesmata has been shown in Arabidopsis root hairs to be dependent of the actomyosin system (Veerabagu et al., 2020).
LDs are also present in roots
LDs can be transiently present in non-dormant vegetative tissues such as leaves or roots, depending on developmental stage and physiological state of the plant (Zienkiewicz and Zienkiewicz, 2020). Yellow nutsedge (Cyperus esculentus) is one of the rare plant to accumulate oil in underground tissue (Linssen et al., 1989), and LDs have been shown to accumulate during C. esculentus tuber development (Turesson et al., 2010). Comparative proteomic analysis of yellow and purple nutsedge (Cyperus rotundus) the closest known relative, whose tubers do not accumulate oil, has revealed that indeed a seed-like proteome is present in yellow but not purple nutsedge (Niemeyer et al., 2022). In fact, the proteome of LD enriched fraction from yellow nutsedge tubers share similarities with the proteome of Arabidopsis seed LDs, notably with the presence of oleosins, steroleosins and SEED LIPID DROPLET PROTEIN (SLDP), that are specifically detected in seed but not leaf LDs (Niemeyer et al., 2022). Because yellow but not purple nutsedge tubers are desiccation-tolerant, it was proposed that oil accumulation probably allows tubers to withstand extended periods of severe water loss. Hensel (1986) has observed the presence of numerous LDs in statocytes of untreated cress roots. In Arabidopsis thaliana, it was shown that disruption of AtSDP1, the lipase involved in seed TAG hydrolysis, leads to the presence of abundant LDs in roots (Kelly et al., 2013). TAG content in roots of the mutant sdp1 increases with the age of the plant and can reach more than 1% of dry weight at maturity, a 50-fold increase over the wild type. Such TAG accumulation in sdp1 roots requires both DGAT1 and PDAT1 and can also be strongly stimulated by the provision of exogenous sugar. However, the physiological role of TAG in sdp1 roots and of LDs in cress statocytes is unknown.
LDs in leaves play different functions
LD abundancy in leaves depends on the diurnal cycle and the physiological stage of the leaf
As for roots, leaves contain limited amount of TAGs under standard conditions, and LDs were rarely described in leaves until the last decade. Yet, in 2006, Lersten and collaborators (Lersten et al., 2006) provided an overview of relevant literature mentioning the observation of LDs in mesophyll cells as early as 1863. The authors completed this review by searching for the presence of LDs in freehand sections of fresh leaves from more than 300 different species. They observed one or several LDs in leaves of 23% of the surveyed species, evenly distributed within the plant kingdom. Gidda et al. (2016) have shown that the abundancy of LDs in leaves varies during diurnal cycling, with a peak of accumulation at the end of the night. It is thus likely that in most of the species from Lersten’s study, LDs could have been observed at another time of the day, and it is reasonable to suggest that LDs are potentially present in leaves of every plant species, depending on the diurnal cycle and the physiological state of the plant. Leaf LD proteome has a particular composition (Brocard et al., 2017; Fernández-Santos et al., 2020; Doner et al., 2021) compared to the seed LD one. In particular, the main seed LD proteins, oleosins, are absent from leaf LDs, while CLO3 and LDAPs are the two most abundant proteins. The presence of LDAP genes in the whole land plant kingdom (de Vries and Ischebeck, 2020) is consistent with the hypothesis that LDs may accumulate in leaves of every land plants. LDs accumulate in senescing leaves (Lersten et al., 2006; Brocard et al., 2017; Zhang et al., 2020; Cohen et al., 2022) where they are supposed to sequester galactolipid-derived FAs before conversion into phloem-mobile sucrose (Kaup et al., 2002). TAG synthesis has been demonstrated to occur in leaves, yet only a very limited TAG content was detected in Arabidopsis senescent leaves (Slocombe et al., 2009; Yang and Ohlrogge, 2009), suggesting this TAG accumulation is transient. It is possible that the abundant LDs observed in senescent leaves have in fact a neutral lipid composition enriched in sterol esters and depleted of TAGs, but this remains to be thoroughly studied.
LDs are involved in stomatal aperture and stomatal development
Stomata, pores at the leaf surface that regulate gas exchanges, have been described to contain LDs in their guard cells but also in the subsidiary ones (McLachlan et al., 2016; Pautov et al., 2016; Pautov et al., 2018; Pautov et al., 2022). The disruption of CLO3 perturbs the opening of Arabidopsis stomata (Aubert et al., 2010), which suggests a role of LDs in the regulation of stomata opening. In fact, LD abundancy correlates with stomatal movements (McLachlan et al., 2016; Pautov et al., 2022) and large LDs in subsidiary cells have been proposed to participate to the turgor pressure and prevent hydropassive stomatal movements (Pautov et al., 2018). McLachlan et al. (2016) have shown that LDs are less abundant in open stomata than in closed ones, and that the regulation of this abundancy is blue light dependent. In the same study, they observed that stomata of mutants impaired in TAG breakdown (sdp1, pxa1 and cgi- (comparative gene identifier-) 58) show decreased light-induced opening and conclude that LDs provide the energy necessary for the stomatal opening via the TAG breakdown and the β-oxidation pathway. Stomatal opening is also triggered by heat stress. It has recently been shown that this regulation requires an increase in TAG synthesis and turnover (Korte et al., 2023), suggesting that LD dynamics are important for efficient stomatal opening not only at dawn, but also in response to heat stress. Measurement of LD abundancy in guard cells in response to heat will be needed to confirm this hypothesis.
In addition to playing a role in the regulation of the stomatal aperture, LDs are also involved in the stomatal development. Ge et al. (2022) observed that LDs are abundant in the meristemoid cell that is at the origin of the stomata, and that their abundancy decreases with stomatal maturation. They have shown that LD dynamics is critical for the stomata development. RABC1, a RAB GTPase involved in stomata morphogenesis that locates at the ER, is targeted to LDs when both LD biogenesis is induced and RABC1 activated by a GUANINE NUCLEOTIDE EXCHANGE FACTOR protein (GEF). The inactivation of RABC1, by the mutation of either RABC1 or RABC1GEF1 genes leads to a defect in stomatal morphology and function, linked with the presence of aberrant large LDs in guard cells. RABC1 regulates the localization of SEIPIN2/3 to LDs, and the seipin2 seipin3 double mutant also display large LDs in deformed guard cells. It is proposed that LDs provide neutral lipids, which mobilization is necessary for guard cell formation (Ge et al., 2022).
LDs represent specialized storing compartments for non-TAG neutral lipids
In latex producing plants, such as rubber trees (Hevea brasiliensis) and rubber dandelion (Taraxacum brevicorniculatum), latex accumulates in rubber particles, organelles with a structure similar to LDs. The hydrophobic core of rubber particles is mainly composed of poly(cis-1,4-isoprene) delimited by a monolayer of phospholipids (Cornish, 2001) associated with at least two major proteins, the small rubber particle proteins and the rubber elongation factor (Dennis and Light, 1989; Oh et al., 1999). These two proteins being homologs to the LDAPs (Gidda et al., 2013; Berthelot et al., 2014), it seems reasonable to postulate that rubber particles are LDs specialized in the storage of latex. Yet, it is still unclear whether the formation of rubber particles occurs at the ER, similarly to the biogenesis of TAG-containing LDs (Dai et al., 2013; Guzha et al., 2023).
Jojoba (Simmondsia chinensis) is a peculiar plant that stores up to 60% of seed weight of wax esters instead of TAGs (Miwa, 1971). These wax esters, composed of a long-chain fatty acid esterified to a long-chain fatty alcohol, accumulate in cotyledons in what was first called wax bodies (Rost and Paterson, 1978). There are hydrolyzed after seed germination to provide energy through the sequential action of a wax ester hydrolase, a fatty alcohol oxidase and a fatty aldehyde dehydrogenase to convert fatty alcohols to fatty acids before β-oxidation (Rajangam et al., 2013). In addition to oleosin and caleosin proteins, which are classically found in seed LDs, LDAP1 has also been identified in LDs from Jojoba seeds (Sturtevant et al., 2020). This suggests a peculiar role of LDAP1 in ensuring correct packaging of particular hydrophobic compounds such as wax esters in Jojoba seeds or isoprenoids in rubber plants because LDAP proteins are usually absent from TAG accumulating seeds.
The neutral lipid core of LDs provides favorable storage site for fat-soluble vitamins. Vitamin E and vitamin A accumulate in LDs from animal cells (Welte and Gould, 2017). In plants, vitamin E and carotenoids (the precursors of vitamin A) are described to accumulate in plastoglobules inside plastids rather than in cytosolic LDs (Deruere et al., 1994; Vidi et al., 2006; Zita et al., 2022). Some studies reported the presence of tocopherols in seed LDs (Zaaboul et al., 2018; Lopez et al., 2023). It remains to be determined with precision whether plant cytosolic LDs contain other vitamins and which ones. Additional non-polar compounds probably accumulate within different peculiar plant species LDs, such as, for example, triterpenoid esters in LDs from tea leaves (Zhou et al., 2019).
LDs participate to the plant response to abiotic and biotic environmental stresses
The implication of LDs in stomata aperture is an illustration of the role played by LDs in plant response to stress. In fact, LDs have been proposed to represent a general mechanism developed not only by land plants, but shared across the green lineage, for stress resilience (de Vries and Ischebeck, 2020). In the following paragraphs, we aim at illustrating such involvement of LDs in plant response to stress.
LDs accumulate in response to some abiotic stresses: the example of thermal stresses
The fluidity of the lipid bilayer of cell membranes is altered by temperature. In response to diurnal and seasonal temperature fluctuations, plants compensate for membrane fluidity imbalance by decreasing or increasing membrane lipid unsaturation degree in response to high or low temperature stress, respectively. Additional membrane alterations occur under changing temperature conditions. Heat stress induces the production of reactive oxygen species, which promote membrane lipid peroxidation (Farmer and Mueller, 2013; Pospisil, 2016). Freezing stress generates intracellular ice, which results in severe dehydration and membrane deformations causing leakiness and loss of bilayer structure (Uemura et al., 1995). Temperature stresses induce transcriptional and metabolic changes important for long-term plant acclimation (Fowler and Thomashow, 2002; Falcone et al., 2004), but also rapid remodeling of membrane lipids important for basal tolerance (Burgos et al., 2011; Li et al., 2015). However, lipid remodeling does not apply only to membrane lipids but also to reserve lipids, which are consistently accumulated during heat and freezing stresses.
In Arabidopsis, Moellering et al. (2010) showed that freezing induces a 7.5-fold increase of leaf TAGs. They demonstrated that this TAG accumulation depends on the galactolipid remodeling of chloroplast membranes by the galactolipid:galactolipid galactosyltransferase SFR2. SFR2 stands for SENSITIVE TO FREEZING 2, and as this name clearly indicates, Arabidopsis plants carrying mutations in SFR2 are not able to resist freezing and show severe intracellular damage with rupture of chloroplast membranes (Fourrier et al., 2008). SFR2 is localized to the outer chloroplast envelope and catalyzes the conversion of MGDG to di-, tri-, and tetragalactosyldiacylglycerol (DGDG, TGDG, and TeDG, respectively) by transglycosylation, resulting in the concomitant production of DAG. SFR2 deficiency impairs freezing-induced accumulation of TAGs, notably those containing the fatty acid C16:3, which is predominately esterified to MGDG in Arabidopsis (Moellering et al., 2010). This result indicates that DAG produced by SFR2 from MGDG is further acylated to TAG. The enzyme catalyzing the conversion of DAG to TAG upon cold exposure has been identified as DGAT1 in both Arabidopsis and Boechera stricta (Arisz et al., 2018; Tan et al., 2018). Overexpression of AtDGAT1 in Arabidopsis enhances freezing tolerance of seedlings (Arisz et al., 2018). Conversely, Arabidopsis dgat1-deficient mutants exhibit reduced tolerance to chilling (3 weeks at 4°C) or freezing (40 min at -8°C) stresses than the wild type (Tan et al., 2018), demonstrating that the conversion of DAG to TAG by DGAT1 is critical for plant freezing tolerance. Together, SFR2 and DGAT1 are believed to contribute to membrane stabilization during freezing stress by decreasing MGDG, DAG, and PA content. Indeed, these lipids tend to form a nonlamellar HII-type phase promoting the shrinkage of membrane structure and membrane ionic leakage (Du and Benning, 2016). TAGs that are consequently produced from MGDG-derived DAGs are most likely stored in cytoplasmic LDs. Indeed, Gidda et al. (2016) showed that the abundance of LDs increases nearly 10-fold in Arabidopsis leaves after 24h of exposure to 4°C. Transcriptional analyses of Arabidopsis LDAP1, LDAP2, and LDAP3 in response to cold stress suggest that cold-induced LDs are decorated by LDAP1 and LDAP3 but not by LDAP2. However, LD accumulation induced by cold stress is reduced in ldap3 but not ldap1 mutant, suggesting a specific role of LDAP3 in cold-induced LD proliferation (Gidda et al., 2016). Another LD-associated protein, EARLY RESPONSE TO DEHYDRATION ERD7, has been identified for its role in cold stress response (Doner et al., 2021). Arabidopsis lines deficient in ERD7 and its homologs are more susceptible to freezing than the wild type, perhaps due to decreased membrane flexibility (Barajas-Lopez et al., 2021). ERD7 does not regulate LD abundance and morphology under normal growth conditions (Doner et al., 2021). Further investigation will be required to determine the exact role of ERD7, especially during stress conditions.
Heat stress also leads to TAG accumulation in plant tissues, as has been consistently reported in different species (Higashi and Saito, 2019). In Arabidopsis leaves exposed to heat stress at 37°C, TAGs accumulate rapidly in the first 3 h and reach a steady state level after 6 h (Mueller et al., 2015; Yurchenko et al., 2018). Compared to other abiotic stresses such as cold, salt, drought, and high light, heat is the strongest inducer of TAG accumulation after short-term stress exposure (Mueller et al., 2015). While the level of total fatty acids remains unchanged during heat stress in Arabidopsis, indicating that heat-induced TAG accumulation is not driven by massive de novo fatty acid synthesis (Mueller et al., 2015), lipidomic studies revealed significant lipid remodeling. In Arabidopsis leaves and seedlings, a temperature shift from 22°C to 37°C or 45°C for 2 h to 1 day leads to the accumulation of TAG species enriched in polyunsaturated fatty acids (PUFAs), such as TAG 54:7, TAG 54:8, and TAG 54:9 (Higashi et al., 2015; Mueller et al., 2017; Shiva et al., 2020). Similar observations were also reported in wheat and Begonia leaves, and castor bean seedlings (Narayanan et al., 2016; Sun et al., 2022; Zhang et al., 2022). A 2-fold accumulation of TAGs in response to 2-h exposure to 37°C was also reported in Nicotiana tabacum germinating pollen, but heat-induced TAGs in pollen, unlike those in leaves, were enriched in saturated FAs (Krawczyk et al., 2022a). In leaves, the increase of PUFAs in TAGs is paralleled by their decrease in chloroplastic membrane lipids such as MGDG, sulfoquinovosyldiacylglycerol, and phosphatidylglycerol (Higashi et al., 2015). This observation suggests that heat-induced TAGs are mainly derived from chloroplastic 16:3 and 18:3 PUFAs. A nice demonstration of this premise was provided by Mueller et al. (2017) by comparing PUFA levels in TAGs in Arabidopsis fad (fatty acid desaturase) 3 and fad7/8 lines, which are deficient in ER- and plastid-located ω-3 desaturases, respectively. Indeed, heat-induced accumulation of 16:3 and 18:3 PUFA in neutral lipids depends on FAD7/8 desaturases, which produce these trienoic PUFAs in chloroplasts, but not on FAD3 desaturase, which synthetizes them in the ER. Consistently, Higashi et al. (2018) showed that a lipase named HIL1 (HEAT INDUCIBLE LIPASE 1), which catabolizes MGDG to produce 18:3 free FA, is involved in the heat-induced remodeling of MGDG and DGDG, and the concomitant accumulation of PUFA-containing TAGs. The reduction of the heat-induced galactolipid turnover in the hil1 mutant is associated with a higher sensitivity to heat stress, compared to the wild type. They also established that HIL1 expression is induced by heat, and that HIL1 localizes to the chloroplast (Higashi et al., 2018). Thus, the first committed step of chloroplastic lipid remodeling induced by heat stress is mainly catalyzed by HIL1, without excluding a minor contribution of other enzymes such as SFR2, as suggested by Mueller et al. (2017). Ultimately, FAs released from chloroplastic membranes are incorporated into cytosolic TAGs and accumulated in LDs. The PHOSPHOLIPID : DIACYLGLYCEROL ACYLTRANSFERASE PDAT1 is required for TAG accumulation in response to heat stress, but DGAT1, the PHOSPHATIDYLCHOLINE : DIACYLGLYCEROL CHOLINE-PHOSPHOTRANSFERASE PDCT, and PHOSPHATIDIC ACID PHOSPHOHYDROLASES PAH1/PAH2 are not (Mueller et al., 2017). PDAT1 is also involved in heat-induced TAG accumulation in guard cells (Korte et al., 2023). Thus, it appears that PUFAs released from chloroplast membranes are mainly channeled into phosphatidylcholine before being sequestered into TAGs. Interestingly, pdat1 seedlings are more sensitive to heat stress, indicating that PDAT1-mediated TAG accumulation is important for plant tolerance to high temperature (Mueller et al., 2017). Heat-induced TAGs accumulate predominantly in cytosolic LDs in Arabidopsis and castor bean (Mueller et al., 2015; Zhang et al., 2022). Consistently, Gidda et al. (2016) reported massive proliferation of LDs after 1-h exposure at 37°C. They also showed that LDAP1 expression is induced in response to heat, and LD proliferation in ldap1 mutant is reduced, suggesting a role of LDAP1 in the LD dynamics induced by heat (Gidda et al., 2016).
Plants accumulate TAGs and LDs in response to other various abiotic stresses such as salinity, drought, high-light, starvation, etc., but the role of neutral lipids in general, and of LDs in particular, is far less documented in these stresses. Plants share common responses against drought and salt stresses since they both induce a severe dehydration (for review Krasensky and Jonak, 2012). A 2.5-fold increase of TAG has been reported when 2 week old Arabidopsis seedlings were submitted either to a 2h drought or 125 mM NaCl treatment for 2h (Mueller et al., 2015). This increase in TAG content is paralleled by a significant increase of the LD number in leaves (Doner et al., 2021). Similarly, an increase of LD abundancy was observed in mesophyll cells of the rocket Eruca sativa after a saline stress (Corti et al., 2023). Several proteins associated with lipid synthesis have been identified in the LD proteome of microalgae Parachlorella kessleri submitted to a salt stress (You et al., 2019). Specifically, an acetyl-CoA carboxylase, LPAT, 3-ketoacyl-CoA synthase, LACS and diacylglycerol kinase were identified. These findings suggest that LDs play a role in the synthesis of phospholipids and membrane remodelling in response to salt stress, at least in algae. A proteomic study (Doner et al., 2021) performed on LDs purified from drought-stressed Arabidopsis leaves, showed an enrichment of LD proteins that have been previously associated to the plant response to stress, such as CLO3, LDAP1, LDAP3, DOX1 and ERD7. CLO3, also named RESPONSIVE TO DESICCATION 20 (RD20), has previously been shown to play key function in the plant response to diverse stresses (Blée et al., 2014). Notably the knock out mutant rd20 is less resistant to drought stress probably because of its alteration of stomatal aperture (Aubert et al., 2010). Similar to CLO3, LDAP gene expression is induced by drought stress (Aubert et al., 2010; Kim E, et al., 2016). Arabidopsis ldap1 and ldap3 mutants are less tolerant to drought stress while the overexpression of some of the Arabidopsis or Taraxacum brevicorniculatum LDAP/SRPP genes confers higher resistance to Arabidopsis plants (Kim E, et al., 2016; Laibach et al., 2018). It is not clear whether the impact of LDAP expression on the plant drought tolerance is due to a direct role of the LDAP proteins in induction of drought tolerance or is a consequence of their involvement in the LD biogenesis and LD size regulation.
LDs in the context of plant-pathogen interactions: friend or foe?
Examples of an involvement of LDs in plant response to pathogens are really scarce, but the expression of several LD-related genes such as LDAP1, CLO3 or α-DOX1 is upregulated in Arabidopsis leaves infected by the fungi Botritys cinerea (Sham et al., 2014), which suggests that LD biosynthesis is induced either by the fungi for hijacking of the host lipid metabolism, or by the plant as a defense against the infection. Indirect evidences of LD accumulation in response to pathogens have also been obtained by following the content of neutral lipids, which are stored mainly within LDs. Recently, an important increase of TAG content (more than 6 fold higher compared to the control plant) was observed in Arabidopsis roots inoculated with the fungi Verticillium longisporum (Schieferle et al., 2021), and also in distal leaves of the same infected plants despite the absence of the fungi in these leaves. This suggests the existence of a systemic signal inducing TAG synthesis in leaves. The bacteria Pseudomonas syringae also induces an increase of TAG content in infected leaves (Zoeller et al., 2012; Schieferle et al., 2021). Interestingly, while the FA composition of TAGs synthesized in response to P. syringae infection clearly suggests that the TAGs derive from the membrane degradation locally provoked by the pathogen, similarly to the process generally described upon abiotic stress, the composition of TAGs accumulating in leaves in response to V. longisporum is peculiar, with fewer insaturations. This could result from the involvement of an alternative TAG synthesis pathway, but this remains to be elucidated. Indeed, the study of dgat1 and pdat1 mutants suggests that TAGs induced by V. longisporum are produced independently of these two acyl-transferases. In animal cells, LDs have been demonstrated to be hijacked by some bacteria, for example Mycobacterium or Chlamydia, to serve as major source of energy and carbon (reviewed in Roingeard and Melo, 2017). But LDs are also described as forming the “first-line of intracellular defense” against some bacteria in mammalian cells by establishing contacts with bacteria and providing antimicrobial peptides (Bosch et al., 2020). Whether the TAG accumulation in Arabidopsis roots and leaves in response to V. longisporum is induced by the fungi to divert the cell metabolism, or by the plant cell as a defense response still needs to be investigated.
Hara-Nishimura and colleagues have demonstrated the involvement of LDs in the production of antifungal molecules such as oxylipins (Shimada et al., 2014). The authors first determined that LDs accumulating in leaves upon infection by the pathogenic fungus Colletotrichum higginsianum contain CLO3 and the α-DIOXYGENASE α-DOX1. Then, CLO3 and α-DOX1 were shown to interact and to convert C18:3 fatty acid into oxylipin 2-hydroxy-octadecatrienoic acid, which has antifungal properties. Later, PAD3, an enzyme involved in the synthesis of the antimicrobial phytoalexin camalexin was also demonstrated to relocate to LDs upon infection by P. syringae (Fernández-Santos et al., 2020). Taken together, these results suggest that LDs can play a role in plant defense as “subcellular factories” producing antimicrobial compounds, at least in Arabidopsis leaves.
The work of Coca and San Segundo (2010) on the CALCIUM DEPENDENT PROTEIN KINASE 1 (CPK1) also suggested an involvement of LDs in the plant response to biotic stress. Arabidopsis cpk1 knock out mutants are indeed more sensible to fungi while CPK1 overexpressors are more resistant. The localization of CPK1 in LDs in addition to peroxisomes, thus suggests a role of LDs in resistance to pathogen, albeit the function of the association of CPK1 with LDs is still obscure.
In contrast, Yang et al. (2021) have shown that the oomycete Phytophtora infestans induces the degradation of LDs in guard cells in order to induce and maintain stomatal opening of potato leaves, thus facilitating the emergence of sporangiophores from opened stomata.
As obligatory parasites, viruses have developed tactics to reroute host cellular functions for their own benefits. In particular, animal (+) RNA viruses harness lipids and manipulate cell membranes to create membranous compartments called viral replication compartments (VRCs) (Zhang et al., 2019). In particular, Laufman et al. (2019) have nicely described the LD hijacking by poliovirus: poliovirus recruits LDs to the forming viral organelles to enable transfer of FAs originating from the lipolysis of LD-stored triacylglycerol to VRCs, where they are incorporated as polar lipids. LD recruitment was shown to be driven by viral proteins that tether the VRCs to the LDs while other viral proteins interact with the host lipolysis machinery to channel FAs into the phospholipids that constitute the VRC membranes. The inhibition of the contact sites formation between LDs and VRCs impedes poliovirus replication (Laufman et al., 2019). Similarly to animal ones, plant RNA viruses have the capacity to induce the proliferation of host endomembranes derived from ER, chloroplasts, mitochondria, peroxisomes, or vacuole, for VRC formation (Jin et al., 2018; Medina-Puche, 2019). Interestingly Tomato bushy stunt virus (TBSV), which is one of the rare plant (+) RNA viruses that can multiply in the model host yeast (Nagy et al., 2016), was shown to replicate faster in the yeast pah1 mutant strain in which the PHOSPHATIDATE PHOSPHATASE PAH1 gene is deleted (Chuang et al., 2014). In addition, TBSV accumulation was hindered in N. benthamiana plants overexpressing the Arabidopsis PAH2 gene. PAH enzymes play key roles in cellular decisions about membrane biogenesis versus lipid storage, thus these results emphasize the importance of the host lipid metabolism regulation by some plant viruses for their replication. Yet to our knowledge, no data are available demonstrating any links between LDs and plant virus infection, and the possible involvement of LDs in plant virus infection, either at the benefit of the virus or as a plant defense mechanism against the virus, remains to be explored.
Diverse pathways participate to LD biogenesis in response to environmental stress
As mentioned above, during environmental stresses like nutrient starvation, or heat, LDs accumulate in cells to later disappear upon favorable environmental conditions. Besides the peculiar role of LDs as reservoir for the oxylipins defense compounds (Shimada et al., 2014), the origin and the exact function of this LD transient formation upon stress is still unclear. The process leading to TAG accumulation seems to differ depending on the stress type, since heat, drought and salt stress induce a rapid TAG accumulation, while TAG synthesis is induced only after several days of exposure to cold, high light or osmotic stress (Mueller et al., 2015). Under oxidative stress conditions, reactive oxygen species are produced, inducing loss of the membrane bilayer integrity, deterioration of the organelles, and leading to generation of cytotoxic free FAs. These FAs released from the membranes are then packed as TAGs and sterol esters in LDs to prevent free FA-induced toxicity (Fan et al., 2013; Xu and Shanklin, 2016). In Arabidopsis plants grown under standard conditions, the disruption of PDAT1 in the tgd1 (trigalactosyldiacylglycerol 1) mutant causes severe decreases in TAG levels with concomitant increases of the content of free FAs, DAG and membrane phospholipids, and premature cell death in growing leaves and floral organs (Fan et al., 2013). This demonstrates the crucial role of TAG biosynthesis and storage for lipid homeostasis and free FA detoxification. Mueller et al. (2017) have shown that PDAT1 but neither DGAT1, nor PDCT, and nor PAH1/PAH2, is required for TAG accumulation in response to heat stress. This confirms that TAGs accumulated under heat stress do not originate from the Kennedy pathway but rather derived from lipid membrane remodeling and FA recycling. Indeed, under stress conditions, the concentration of toxic free FAs is highly regulated by feedback inhibition of FA biosynthesis by oleic acid-ACYL CARRIER PROTEIN (18:1-ACP), which inhibits plastidic ACETYL-COA CARBOXYLASE (Flügge et al., 2011). In contrast to heat stress, upon freezing, DGAT1 is an essential player of TAG formation (Arisz et al., 2018). During cold acclimation phase, TAG accumulates in B. stricta seedlings from conversion either of phosphatidylcholine or of MGDG, depending on the ecotypes, while some TAGs are formed with newly synthesized FAs (Arisz et al., 2018).
LD degradation probably participate to plant stress recovery upon return to normal environmental conditions
Plant tolerance to stress depends not only on the efficient elimination of damaged organelles/membranes, but also on the capacity to remodel functional compartments upon return to normal conditions. Recent studies have revealed immediate rapid disappearance of LDs post-stress (Mueller et al., 2015; Graef, 2018; Zienkiewicz and Zienkiewicz, 2020). Thus, it has been postulated that LD degradation is involved in stress recovery upon return to normal environmental conditions. The essential role of LDs for the resumption of cell growth is documented in yeast and microalgae (Graef, 2018; Lee et al., 2020; Zienkiewicz and Zienkiewicz, 2020), and the mobilization of seed LDs during post-germinative growth is well described (Kelly et al., 2011; Deruyffelaere et al., 2015; Thazar-Poulot et al., 2015; D’Andrea, 2016; Deruyffelaere et al., 2018). However, the mechanisms involved in the plant recovery phase after a period of stress, and leading to a rapid built of new organelle membranes from damaged ones, are still an enigma. In particular, it is unclear whether FAs transiently stored as TAGs in LDs are hydrolyzed during stress recovery for energy supply or recycled as source of FAs for lipid synthesis to restore the integrity of membranes. Probably both processes happen.
Under deprivation of nutrients, which induces growth arrest, LD accumulation allows the storage of the excess carbon produced by photosynthesis, and will provide energy and carbon to quickly restart when the conditions get better. Fan et al. (2019b) have suggested that under starvation conditions, the degradation of membrane lipids and the subsequent formation of TAGs in leaves may participate to the maintenance of energy homeostasis.
Very little is known about the degradation of plant LDs after stress and the lipid metabolism associated to post-stress recovery. Mueller et al. (2015) reported that TAG mobilization occurs in less than one day upon recovery from heat stress suggesting a rapid and acute degradation of stress-induced TAGs during the recovery phase. Lipids stored in LDs are degraded by two distinct pathways: lipolysis which relies on the action of lipases, and lipophagy, a specialized form of autophagy (Figure 2).
Lipolysis, coupled to β-oxidation, is the main pathway that supply energy and carbon for seedling growth after germination (Kelly et al., 2011; Thazar-Poulot et al., 2015). So far, the best characterized TAG lipases directly involved in LD mobilization in plants are SDP1 and SDP1-L proteins (Eastmond, 2006; Quettier and Eastmond, 2009; Kelly et al., 2011). Previous studies have shown that the expression level of AtSDP1 increases during natural leaf senescence (Troncoso-Ponce et al., 2013) and that disruption of AtSDP1 leads to TAG accumulation also in vegetative tissues, such as leaves, stems, and roots (Kelly et al., 2013; Fan et al., 2014). Later, SDP1-dependent lipolysis was proposed to participate in leaf TAG mobilization from dark-induced LDs (Fan, 2017). AtCGI-58 transcript levels are up-regulated during leaf senescence (Troncoso-Ponce et al., 2013), and atcgi-58 mutant shows a significant increase in TAG content of leaf mesophyll cells (James et al., 2010). Similarly to mammalian CGI-58 that has been shown to regulate TAG breakdown by activating ATGL (Lass et al., 2006), Arabidopsis CGI-58 has been suggested to promote TAG turn-over by activating PXA1 hydrolysis activity (Park et al., 2013). Many genes putatively coding for lipases are expressed during leaf senescence (Troncoso-Ponce et al., 2013), suggesting that more lipases could be involved in LD turnover during leaf senescence or in response to environmental stress, yet their activity remains to be described.
Post-germinative LD degradation is delayed in LDAP1-deficient Arabidopsis plants (Gidda et al., 2016), suggesting that LDAP1 (and possibly other LDAPs) might orchestrate the LD docking and activation of actors of LD/TAG degradation, similar to the function of PERILIPINs (PLINs) in mammalian cells (D’Andrea, 2016). Moreover, LDAPs are differentially up-regulated by diverse stresses and are associated with a better tolerance to drought (Gidda et al., 2013; Kim et al., 2016a). On the basis of these observations, we anticipate that LDAPs might be involved in LD remobilization and plant fitness upon stress recovery not only via their structural function on LDs, but also by promoting the formation and/or recruitment of protein modules dedicated to this mobilization.
In addition to lipolysis, lipophagy is the alternative LD degradation pathway during which LDs are specifically targeted by the autophagy machinery, as reported in mammals, yeast and algae (Singh et al., 2009; Zhao et al., 2014; Graef, 2018). Two distinct types of autophagy have been described in plants: macroautophagy and microautophagy (Figure 2). Macroautophagy involves the formation in the cytosol of double membrane structures called autophagosomes (Yoshimoto and Ohsumi, 2018). During their formation, autophagosomes entrap different cargo and carry them to the lytic vacuole lumen where they will be released after fusion of the autophagosome outer membrane with the tonoplast. Microautophagy is characterized by the invagination of the tonoplast to trap cytoplasmic material and create autophagic bodies within the vacuole lumen (Masclaux-Daubresse et al., 2020). Diverse ATG proteins participate in the induction of macro- and micro-autophagy. Plant autophagy is highly induced by various abiotic stresses, including nutrient deprivation (Janse van Rensburg et al., 2019), dark-induced starvation (Fan et al., 2019b), drought (Bao et al., 2020), and biotic stresses as pathogen infection (Dagdas et al., 2016). Several studies have provided evidence of an important role of autophagy in LDs remobilization in non-seed tissues (Kurusu et al., 2014; Fan et al., 2019a). Potential role of lipophagy in pollen grain maturation was described in rice (Kurusu et al., 2014), where Osatg7 mutant defective in autophagy showed lower contents of TAGs and LDs in mature pollen, but a higher accumulation of LDs in tapetal cells compared to wild type plants. These results, suggest that ATG7 may be responsible for LD degradation and TAG remobilization in the tapetum. More recently, Zhao et al. (2020) showed that silencing of ATG2 and ATG5 leads to a lower number of autophagosomes at the germinative pollen aperture and is accompanied by inhibition of pollen germination and significant accumulation of TAGs and DAGs in pollen grains. Autophagy is also implicated in LD breakdown in senescent leaves of watermelon (Cirullus lanatus) (Zhang et al., 2020) and in LD turnover in Arabidopsis leaves during dark-induced starvation (Fan et al., 2019a). Indeed, ultrastructural analysis performed on dark-treated atsdp1-4 leaves, defective in cytosolic lipolysis, showed accumulation of LDs in the central vacuole, probably resulting from the trapping of LDs by invagination of the tonoplast during microlipophagy (Fan et al., 2019a). In addition, macro-autophagy has been shown to be involved in the degradation of lipids originating from the endomembranes of various organelles, except for those of the chloroplasts (Fan et al., 2019a; Havé et al., 2019). Whether lipophagy occurs in response to other stresses and contribute to plant recovery remains an open question, which requires further investigations.
Conclusion
While described in non-seed tissues since more than a century, the physiological roles of LDs in vegetative cells have been under investigation since only few years. Their presence in almost every organs and tissue of the plant suggest a wider function than their primary role as reservoir of energy for post-germinative growth. In fact, plant LDs must be considered as dynamic versatile organelles with numerous roles. Of course, they principally play functions related to lipid metabolism such as protection against cytotoxicity of free FAs derived from membrane degradation, and remobilization of neutral lipids for membranes synthesis during bud dormancy release or pollen tube growth for example. Yet they also allow the storage of particular lipophilic compounds such as the antifungal oxylipins, isoprenoids in rubber particles and probably some vitamins that still need to be identified in plants. In addition, they probably have cryoprotectant properties in seeds and dormant buds, as well as in roots. Within cells, LDs are highly mobile organelles (reviewed in non-plant cells by Kilwein and Welte, 2019) and share multiple transient contact sites with other organelles such as the ER and peroxisomes of course, but also mitochondria, vacuole, plasma membrane (reviewed in Scholz et al., 2022) and chloroplast (Brocard et al., 2017). The nature of such LD-organelle contacts is not well defined in plant. A continuity between the LD monolayer and the cytosolic leaflet of the ER membrane is described for example in the case of LD-ER connections during LD nucleation and growth. Membrane contact sites (MCS), which are defined as the close (less than 30 nm) apposition of two membranes without any fusion event, mediated by tethering proteins, have recently been proposed to occur between LDs and plasma membrane, through the tethering of SEED LD PROTEIN (SLDP) and LD-PLASMA MEMBRANE ADAPTOR (LIPA) proteins (Krawczyk et al., 2022b). However, the confocal microscopic data provided in this study do not allow to firmly exclude any membrane fusion event and thus to firmly identify sensu stricto MCS at the site of LD-plasma membrane junctions. Similarly, the exact identity of the connections described between LDs and peroxisomes (Reviewed in Esnay et al., 2020) still remains to be determined. Whatever their exact nature and structure, these contacts would favor the necessary exchange of lipids (reviewed in Michaud and Jouhet, 2019; Scholz et al., 2022) occurring under stress condition, for example for membrane remodeling (cf. Figure 2). In addition, such contacts would also allow the transfer of enzymes and other hydrophobic proteins that would more easily transit within the cell by riding piggyback on LDs than through the aqueous cytosol. Given the high number of proteins identified in LD proteomes, different additional functions of plant LDs most probably remain to be discovered. Notably, involvement in resistance to ER stress or to mitochondrial damage during autophagy, that have been described in animal cells, are also presumably supported by LDs in plant cells.
Nowadays, LDs are the target of choice for researchers and industrialists to modify the lipid metabolism of plants for biotechnological purposes (e.g. increase of oil content, production of high-value proteins requiring hydrophobic environment). However, the involvement of LDs in so many physiological functions of the plant should be taken into account during such studies in order to minimize the impact on the development (and thus the yield) of the plant.
Author contributions
Each author participated to the writing of this review. All authors contributed to the article and approved the submitted version.
Funding
This work was supported by the French Agency for Research (Agence Nationale de la Recherche, grant number ANR-21-CE20-0013 RecovOil Project).
Acknowledgments
Authors sincerely thank Dr. J. Joubès and Dr. L. Brocard for providing micrography of Arabidopsis pollen grain. The authors apologize to all the authors whose work could not be cited in this review due to space limitations. Authors thanks the CNRS (Centre National pour la Recherche Scientifique) for providing the necessary facilities. Cell imagery was performed on the plant imagery platform of the Bordeaux Imaging Center (BIC), which belongs to the national research infrastructure France BioImaging.
Conflict of interest
The authors declare that the research was conducted in the absence of any commercial or financial relationships that could be construed as a potential conflict of interest.
Publisher’s note
All claims expressed in this article are solely those of the authors and do not necessarily represent those of their affiliated organizations, or those of the publisher, the editors and the reviewers. Any product that may be evaluated in this article, or claim that may be made by its manufacturer, is not guaranteed or endorsed by the publisher.
References
Arisz, S. A., Heo, J.-Y., Koevoets, I. T., Zhao, T., van Egmond, P., Meyer, A. J., et al. (2018). DIACYLGLYCEROL ACYLTRANSFERASE1 contributes to freezing tolerance. Plant Physiol. 177, 1410–1424. doi: 10.1104/pp.18.00503
Arzac, M. I., Fernández-Marín, B., García-Plazaola, J. I. (2022). More than just lipid balls: quantitative analysis of plastoglobule attributes and their stress-related responses. Planta 255, 62. doi: 10.1007/s00425-022-03848-9
Aubert, Y., Vile, D., Pervent, M., Aldon, D., Ranty, B., Simonneau, T., et al. (2010). RD20, a stress-inducible caleosin, participates in stomatal control, transpiration and drought tolerance in arabidopsis thaliana. Plant Cell Physiol. 51, 1975–1987. doi: 10.1093/pcp/pcq155
Avin-Wittenberg, T., Bajdzienko, K., Wittenberg, G., Alseekh, S., Tohge, T., Bock, R., et al. (2015). Global analysis of the role of autophagy in cellular metabolism and energy homeostasis in arabidopsis seedlings under carbon starvation. Plant Cell 27, 306–322. doi: 10.1105/tpc.114.134205
Bao, Y., Song, W.-M., Wang, P., Yu, X., Li, B., Jiang, C., et al. (2020). COST1 regulates autophagy to control plant drought tolerance. Proc. Natl. Acad. Sci. U S A 117, 7482–7493. doi: 10.1073/pnas.1918539117
Barajas-Lopez, J., de, D., Tiwari, A., Zarza, X., Shaw, M. W., Pascual, J. S., et al. (2021). EARLY RESPONSE TO DEHYDRATION 7 remodels cell membrane lipid composition during cold stress in arabidopsis. Plant Cell Physiol. 62, 80–91. doi: 10.1093/pcp/pcaa139
Baud, S., Dichow, N. R., Kelemen, Z., d’Andréa, S., To, A., Berger, N., et al. (2009). Regulation of HSD1 in seeds of arabidopsis thaliana. Plant Cell Physiol. 50, 1463–1478. doi: 10.1093/pcp/pcp092
Berggren, B. (1984). Ulfrastructure of the histological zones in growing vegetative buds of salix spp. Nord J. Bot. 4, 771–790. doi: 10.1111/j.1756-1051.1984.tb02009.x
Berthelot, K., Lecomte, S., Estevez, Y., Zhendre, V., Henry, S., Thévenot, J., et al. (2014). Rubber particle proteins, HbREF and HbSRPP, show different interactions with model membranes. biochim. biophys. Acta BBA - Biomembr 1838, 287–299. doi: 10.1016/j.bbamem.2013.08.025
Blée, E., Boachon, B., Burcklen, M., Guédard, M. L., Hanano, A., Heintz, D., et al. (2014). The reductase activity of the arabidopsis caleosin RESPONSIVE TO DESSICATION20 mediates gibberellin-dependent flowering time, abscisic acid sensitivity, and tolerance to oxidative stress. Plant Physiol. 166, 109–124. doi: 10.1104/pp.114.245316
Bosch, M., Sánchez-Álvarez, M., Fajardo, A., Kapetanovic, R., Steiner, B., Dutra, F., et al. (2020). Mammalian lipid droplets are innate immune hubs integrating cell metabolism and host defense. Science 370. doi: 10.1126/science.aay8085
Bréhélin, C., Kessler, F., Vanwijk, K. (2007). Plastoglobules: versatile lipoprotein particles in plastids. Trends Plant Sci. 12, 260–266. doi: 10.1016/j.tplants.2007.04.003
Brocard, L., Immel, F., Coulon, D., Esnay, N., Tuphile, K., Pascal, S., et al. (2017). Proteomic analysis of lipid droplets from arabidopsis aging leaves brings new insight into their biogenesis and functions. Front. Plant Sci. 8. doi: 10.3389/fpls.2017.00894
Burgos, A., Szymanski, J., Seiwert, B., Degenkolbe, T., Hannah, M. A., Giavalisco, P., et al. (2011). Analysis of short-term changes in the Arabidopsis thaliana glycerolipidome in response to temperature and light. Plant J. 66, 656–668. doi: 10.1111/j.1365-313X.2011.04531.x
Chapman, K. D., Aziz, M., Dyer, J. M., Mullen, R. T. (2019). Mechanisms of lipid droplet biogenesis. Biochem. J. 476, 1929–1942. doi: 10.1042/BCJ20180021
Chapman, K. D., Dyer, J. M., Mullen, R. T. (2012). Biogenesis and functions of lipid droplets in plants:Thematic Review Series: Lipid Droplet Synthesis and Metabolism: from Yeast to Man. J. Lipid Res. 53, 215–226. doi: 10.1194/jlr.R021436
Chapman, K. D., Trelease, R. N. (1991). Acquisition of membrane lipids by differentiating glyoxysomes: role of lipid bodies. J. Cell Biol. 115, 995–1007. doi: 10.1083/jcb.115.4.995
Chen, D.-H., Chyan, C.-L., Jiang, P.-L., Chen, C.-S., Tzen, J. T. C. (2012). The same oleosin isoforms are present in oil bodies of rice embryo and aleurone layer while caleosin exists only in those of the embryo. Plant Physiol. Biochem. 60, 18–24. doi: 10.1016/j.plaphy.2012.07.022
Choi, Y. J., Zaikova, K., Yeom, S.-J., Kim, Y.-S., Lee, D. W. (2022). Biogenesis and lipase-mediated mobilization of lipid droplets in plants. Plants 11, 1243. doi: 10.3390/plants11091243
Chuang, C., Barajas, D., Qin, J., Nagy, P. D. (2014). Inactivation of the host lipin gene accelerates RNA virus replication through viral exploitation of the expanded endoplasmic reticulum membrane. PloS Pathog. 10, e1003944. doi: 10.1371/journal.ppat.1003944
Coca, M., San Segundo, B. (2010). AtCPK1 calcium-dependent protein kinase mediates pathogen resistance in arabidopsis. Plant J. Cell Mol. Biol. 63, 526–540. doi: 10.1111/j.1365-313X.2010.04255.x
Cohen, M., Hertweck, K., Itkin, M., Malitsky, S., Dassa, B., Fischer, A. M., et al. (2022). Enhanced proteostasis, lipid remodeling, and nitrogen remobilization define barley flag leaf senescence. J. Exp. Bot. 73, 6816–6837. doi: 10.1093/jxb/erac329
Coleman, R. A. (2020). The “discovery” of lipid droplets: a brief history of organelles hidden in plain sight. Biochim. Biophys. Acta BBA - Mol. Cell Biol. Lipids 1865, 158762. doi: 10.1016/j.bbalip.2020.158762
Cornish, K. (2001). Similarities and differences in rubber biochemistry among plant species. Phytochemistry 57, 1123–1134. doi: 10.1016/S0031-9422(01)00097-8
Corti, E., Falsini, S., Schiff, S., Tani, C., Gonnelli, C., Papini, A. (2023). Saline stress impairs lipid storage mobilization during germination in eruca sativa. Plants 12, 366. doi: 10.3390/plants12020366
Coulon, D., Brocard, L., Tuphile, K., Bréhélin, C. (2020). Arabidopsis LDIP protein locates at a confined area within the lipid droplet surface and favors lipid droplet formation. Biochimie 169, 29–40. doi: 10.1016/j.biochi.2019.09.018
Cui, S., Hayashi, Y., Otomo, M., Mano, S., Oikawa, K., Hayashi, M., et al. (2016). Sucrose production mediated by lipid metabolism suppresses the physical interaction of peroxisomes and oil bodies during germination of arabidopsis thaliana. J. Biol. Chem. 291, 19734–19745. doi: 10.1074/jbc.M116.748814
Dagdas, Y. F., Belhaj, K., Maqbool, A., Chaparro-Garcia, A., Pandey, P., Petre, B., et al. (2016). An effector of the Irish potato famine pathogen antagonizes a host autophagy cargo receptor. eLife 5, e10856. doi: 10.7554/eLife.10856
Dai, L., Kang, G., Li, Y., Nie, Z., Duan, C., Zeng, R. (2013). In-depth proteome analysis of the rubber particle of hevea brasiliensis (para rubber tree). Plant Mol. Biol. 82, 155–168. doi: 10.1007/s11103-013-0047-y
D’Andrea, S. (2016). Lipid droplet mobilization: The different ways to loosen the purse strings. Biochimie 120, 17–27. doi: 10.1016/j.biochi.2015.07.010
D’Andréa, S., Canonge, M., Beopoulos, A., Jolivet, P., Hartmann, M. A., Miquel, M., et al. (2007). At5g50600 encodes a member of the short-chain dehydrogenase reductase superfamily with 11beta- and 17beta-hydroxysteroid dehydrogenase activities associated with arabidopsis thaliana seed oil bodies. Biochimie 89, 222–229. doi: 10.1016/j.biochi.2006.09.013
Davidi, L., Katz, A., Pick, U. (2012). Characterization of major lipid droplet proteins from dunaliella. Planta 236, 19–33. doi: 10.1007/s00425-011-1585-7
Dennis, M. S., Light, D. R. (1989). Rubber elongation factor from hevea brasiliensis: identification, characterization, and role in rubber biosynthesis.*. J. Biol. Chem. 264, 18608–18617. doi: 10.1016/S0021-9258(18)51510-6
Deruére, J., Bouvier, F., Steppuhn, J., Klein, A., Camara, B., Kuntz, M. (1994). Structure and expression of two plant genes encoding chromoplast-specific proteins: occurrence of partially spliced transcripts. Biochem. Biophys. Res. Commun. 199, 1144–1150. doi: 10.1006/bbrc.1994.1350
Deruyffelaere, C., Bouchez, I., Morin, H., Guillot, A., Miquel, M., Froissard, M., et al. (2015). Ubiquitin-mediated proteasomal degradation of oleosins is involved in oil body mobilization during post-germinative seedling growth in arabidopsis. Plant Cell Physiol. 56, 1374–1387. doi: 10.1093/pcp/pcv056
Deruyffelaere, C., Purkrtova, Z., Bouchez, I., Collet, B., Cacas, J.-L., Chardot, T., et al. (2018). PUX10 is a CDC48A adaptor protein that regulates the extraction of ubiquitinated oleosins from seed lipid droplets in arabidopsis. Plant Cell 30, 2116–2136. doi: 10.1105/tpc.18.00275
de Vries, J., Ischebeck, T. (2020). Ties between stress and lipid droplets pre-date seeds. Trends Plant Sci. 25, 1203–1214. doi: 10.1016/j.tplants.2020.07.017
Doner, N. M., Seay, D., Mehling, M., Sun, S., Gidda, S. K., Schmitt, K., et al. (2021). Arabidopsis thaliana EARLY RESPONSIVE TO DEHYDRATION 7 localizes to lipid droplets via its senescence domain. Front. Plant Sci. 12. doi: 10.3389/fpls.2021.658961
Du, Z.-Y., Benning, C. (2016). Triacylglycerol accumulation in photosynthetic cells in plants and algae. In: Nakamura, Y., Li-Beisson, Y. eds. Lipids in Plant and Algae Development. Subcellular Biochemistry. (Springer, Cham) 86, 179–205. doi: 10.1007/978-3-319-25979-6_8
Dussert, S., Guerin, C., Andersson, M., Joët, T., Tranbarger, T. J., Pizot, M., et al. (2013). Comparative transcriptome analysis of three oil palm fruit and seed tissues that differ in oil content and fatty acid composition. Plant Physiol. 162, 1337–1358. doi: 10.1104/pp.113.220525
Eastmond, P. J. (2006). SUGAR-DEPENDENT1 encodes a patatin domain triacylglycerol lipase that initiates storage oil breakdown in germinating arabidopsis seeds. Plant Cell 18, 665–675. doi: 10.1105/tpc.105.040543
Eastmond, P. J., Germain, V., Lange, P. R., Bryce, J. H., Smith, S. M., Graham, I. A. (2000). Postgerminative growth and lipid catabolism in oilseeds lacking the glyoxylate cycle. Proc. Natl. Acad. Sci. U A 97, 5669–5674. doi: 10.1073/pnas.97.10.5669
Esnay, N., Dyer, J. M., Mullen, R. T., Chapman, K. D. (2020). Lipid droplet–peroxisome connections in plants. Contact 3, 251525642090876. doi: 10.1177/2515256420908765
Falcone, D. L., Ogas, J. P., Somerville, C. R. (2004). Regulation of membrane fatty acid composition by temperature in mutants of arabidopsis with alterations in membrane lipid composition. BMC Plant Biol. 4, 17. doi: 10.1186/1471-2229-4-17
Fan, J., Yan, C., Roston, R., Shanklin, J., Xu, C. (2014). Arabidopsis lipins, PDAT1 acyltransferase, and SDP1 triacylglycerol lipase synergistically direct fatty acids toward β-oxidation, thereby maintaining membrane lipid homeostasis. Plant Cell 26, 4119–4134. doi: 10.1105/tpc.114.130377
Fan, J., Yan, C., Xu, C. (2013). Phospholipid:diacylglycerol acyltransferase-mediated triacylglycerol biosynthesis is crucial for protection against fatty acid-induced cell death in growing tissues of arabidopsis. Plant J. 76, 930–942. doi: 10.1111/tpj.12343
Fan, J., Yu, L., Xu, C. (2019a). Dual role for autophagy in lipid metabolism in arabidopsis. Plant Cell 31, 1598–1613. doi: 10.1105/tpc.19.00170
Fan, J., Zhou, C., Yu, L., Li, P., Shanklin, J., Xu, C. (2019b). Diversion of carbon flux from sugars to lipids improves the growth of an arabidopsis starchless mutant. Plants 8, 229. doi: 10.3390/plants8070229
Fan, J., Yu, L., Xu, C. (2017). A Central Role for Triacylglycerol in Membrane Lipid Breakdown, Fatty Acid β -Oxidation, and Plant Survival under Extended Darkness. Plant Physiol. 174, 1517–1530. doi: 10/gbkjrr
Farmer, E. E., Mueller, M. J. (2013). ROS-mediated lipid peroxidation and RES-activated signaling. Annu. Rev. Plant Biol. 64, 429–450. doi: 10.1146/annurev-arplant-050312-120132
Fernández-Santos, R., Izquierdo, Y., López, A., Muñiz, L., Martínez, M., Cascón, T., et al. (2020). Protein profiles of lipid droplets during the hypersensitive defense response of arabidopsis against pseudomonas infection. Plant Cell Physiol. 61, 1144–1157. doi: 10.1093/pcp/pcaa041
Flügge, U.-I., Häusler, R. E., Ludewig, F., Gierth, M. (2011). The role of transporters in supplying energy to plant plastids. J. Exp. Bot. 62, 2381–2392. doi: 10.1093/jxb/erq361
Fourrier, N., Bédard, J., Lopez-Juez, E., Barbrook, A., Bowyer, J., Jarvis, P., et al. (2008). A role for SENSITIVE TO FREEZING2 in protecting chloroplasts against freeze-induced damage in Arabidopsis. Plant J. 55, 734–745. doi: 10.1111/j.1365-313X.2008.03549.x
Fowler, S., Thomashow, M. F. (2002). Arabidopsis transcriptome profiling indicates that multiple regulatory pathways are activated during cold acclimation in addition to the CBF cold response pathway. Plant Cell 14, 1675–1690. doi: 10.1105/tpc.003483
Fu, Y. (2015). The cytoskeleton in the pollen tube. Curr. Opin. Plant Biol. 28, 111–119. doi: 10.1016/j.pbi.2015.10.004
Fulda, M., Schnurr, J., Abbadi, A., Heinz, E., Browse, J. (2004). Peroxisomal acyl-CoA synthetase activity is essential for seedling development in arabidopsis thaliana. Plant Cell 16, 394–405. doi: 10.1105/tpc.019646
Ge, S., Zhang, R.-X., Wang, Y.-F., Sun, P., Chu, J., Li, J., et al. (2022). The arabidopsis rab protein RABC1 affects stomatal development by regulating lipid droplet dynamics. Plant Cell 34, 4274–4292. doi: 10.1093/plcell/koac239
Gidda, S. K., Park, S., Pyc, M., Yurchenko, O., Cai, Y., Wu, P., et al. (2016). Lipid droplet-associated proteins (LDAPs) are required for the dynamic regulation of neutral lipid compartmentation in plant cells. Plant Physiol. 170, 2052–2071. doi: 10.1104/pp.15.01977
Gidda, S. K., Watt, S. C., Collins-Silva, J., Kilaru, A., Arondel, V., Yurchenko, O., et al. (2013). Lipid droplet-associated proteins (LDAPs) are involved in the compartmentalization of lipophilic compounds in plant cells. Plant Signal. Behav. 8, e27141. doi: 10.4161/psb.27141
Goold, H., Beisson, F., Peltier, G., Li-Beisson, Y. (2015). Microalgal lipid droplets: composition, diversity, biogenesis and functions. Plant Cell Rep. 34, 545–555. doi: 10.1007/s00299-014-1711-7
Graef, M. (2018). Lipid droplet-mediated lipid and protein homeostasis in budding yeast. FEBS Lett. 592, 1291–1303. doi: 10.1002/1873-3468.12996
Graham, I. A. (2008). Seed storage oil mobilization. Annu. Rev. Plant Biol. 59, 115–142. doi: 10.1146/annurev.arplant.59.032607.092938
Greer, M. S., Cai, Y., Gidda, S. K., Esnay, N., Kretzschmar, F. K., Seay, D., et al. (2020). SEIPIN isoforms interact with the membrane-tethering protein VAP27-1 for lipid droplet formation. Plant Cell 32, 2932–2950. doi: 10.1105/tpc.19.00771
Guan, Y., Meng, X., Khanna, R., LaMontagne, E., Liu, Y., Zhang, S. (2014). Phosphorylation of a WRKY transcription factor by MAPKs is required for pollen development and function in arabidopsis. PloS Genet. 10, e1004384. doi: 10.1371/journal.pgen.1004384
Guzha, A., Whitehead, P., Ischebeck, T., Chapman, K. D. (2023). Lipid droplets: packing hydrophobic molecules within the aqueous cytoplasm. Annu. Rev. Plant Biol. 74, annurev–arplant-070122-021752. doi: 10.1146/annurev-arplant-070122-021752
Guzicka, M., Pawłowski, T. A., Staszak, A., Rożkowski, R., Chmura, D. J. (2018). Molecular and structural changes in vegetative buds of Norway spruce during dormancy in natural weather conditions. Tree Physiol. 38, 721–734. doi: 10.1093/treephys/tpx156
Guzicka, M., Wozny, A. (2003). Mid-winter ultrastructural changes in the vegetative embryonic shoot of Norway spruce [Picea abies (L.) karst.]. Ann. For. Sci. 60, 461–466. doi: 10.1051/forest:2003039
Han, B., Xu, H., Feng, Y., Xu, W., Cui, Q., Liu, A. (2020). Genomic characterization and expressional profiles of autophagy-related genes (ATGs) in oilseed crop castor bean (Ricinus communis l.). Int. J. Mol. Sci. 21, 562. doi: 10.3390/ijms21020562
Hanano, A., Blée, E., Murphy, D. J. (2023). Caleosin/Peroxygenases: multifunctional proteins in plants. Ann. Bot. 131, 387–409. doi: 10.1093/aob/mcad001
Havé, M., Luo, J., Tellier, F., Balliau, T., Cueff, G., Chardon, F., et al. (2019). Proteomic and lipidomic analyses of the arabidopsis atg5 autophagy mutant reveal major changes in endoplasmic reticulum and peroxisome metabolisms and in lipid composition. New Phytol. 223, 1461–1477. doi: 10.1111/nph.15913
Hayashi, Y., Hayashi, M., Hayashi, H., Hara-Nishimura, I., Nishimura, M. (2001). Direct interaction between glyoxysomes and lipid bodies in cotyledons of the arabidopsis thaliana ped1 mutant. Protoplasma 218, 83–94. doi: 10.1007/BF01288364
Hensel, W. (1986). Cytodifferentiation of polar plant cells: use of anti-microtubular agents during the differentiation of statocytes from cress roots (Lepidium sativum l.). Planta 169, 293–303. doi: 10.1007/BF00392123
Hernández, M. L., Lima-Cabello, E., Alché, J., de, D., Martínez-Rivas, J. M., Castro, A. J. (2020). Lipid composition and associated gene expression patterns during pollen germination and pollen tube growth in olive (Olea europaea l.). Plant Cell Physiol. 61, 1348–1364. doi: 10.1093/pcp/pcaa063
Higashi, Y., Okazaki, Y., Myouga, F., Shinozaki, K., Saito, K. (2015). Landscape of the lipidome and transcriptome under heat stress in arabidopsis thaliana. Sci. Rep. 5, 10533. doi: 10.1038/srep10533
Higashi, Y., Okazaki, Y., Takano, K., Myouga, F., Shinozaki, K., Knoch, E., et al. (2018). Heat inducible lipase1 remodels chloroplastic monogalactosyldiacylglycerol by liberating α-linolenic acid in arabidopsis leaves under heat stress. Plant Cell 30, 1887–1905. doi: 10.1105/tpc.18.00347
Higashi, Y., Saito, K. (2019). Lipidomic studies of membrane glycerolipids in plant leaves under heat stress. Prog. Lipid Res. 75, 100990. doi: 10.1016/j.plipres.2019.100990
Horn, P. J., Korte, A. R., Neogi, P. B., Love, E., Fuchs, J., Strupat, K., et al. (2012). Spatial mapping of lipids at cellular resolution in embryos of cotton. Plant Cell 24, 622–636. doi: 10.1105/tpc.111.094581
Huang, A. H. C. (2018). Plant lipid droplets and their associated proteins: potential for rapid advances. Plant Physiol. 176, 1894–1918. doi: 10.1104/pp.17.01677
Huang, S., Liu, Z., Cao, W., Li, H., Zhang, W., Cui, Y., et al. (2022). The plant ESCRT component FREE1 regulates peroxisome-mediated turnover of lipid droplets in germinating arabidopsis seedlings. Plant Cell 34, 4255–4273. doi: 10.1093/plcell/koac195
Ischebeck, T. (2016). Lipids in pollen - they are different. Biochim. Biophys. Acta. 1861, 1315–1328. doi: 10.1016/j.bbalip.2016.03.023
Ischebeck, T., Krawczyk, H. E., Mullen, R. T., Dyer, J. M., Chapman, K. D. (2020). Lipid droplets in plants and algae: distribution, formation, turnover and function. Semin. Cell Dev. Biol. 108, 82–93. doi: 10.1016/j.semcdb.2020.02.014
James, C. N., Horn, P. J., Case, C. R., Gidda, S. K., Zhang, D., Mullen, R. T., et al. (2010). Disruption of the Arabidopsis CGI-58 homologue produces chanarin–dorfman-like lipid droplet accumulation in plants. Proc. Natl. Acad. Sci. 107, 17833–17838. doi: 10.1073/pnas.0911359107
Janse van Rensburg, H. C., Van den Ende, W., Signorelli, S. (2019). Autophagy in plants: both a puppet and a puppet master of sugars. Front. Plant Sci. 10. doi: 10.3389/fpls.2019.00014
Jin, X., Cao, X., Wang, X., Jiang, J., Wan, J., Laliberté, J.-F., et al. (2018). Three-dimensional architecture and biogenesis of membrane structures associated with plant virus replication. Front. Plant Sci. 9. doi: 10.3389/fpls.2018.00057
Jolivet, P., Acevedo, F., Boulard, C., d’Andrea, S., Faure, J. D., Kohli, A., et al. (2013). Crop seed oil bodies: from challenges in protein identification to an emerging picture of the oil body proteome. Proteomics 13, 1836–1849. doi: 10.1002/pmic.201200431
Jolivet, P., Boulard, C., Bellamy, A., Larre, C., Barre, M., Rogniaux, H., et al. (2009). Protein composition of oil bodies from mature brassica napus seeds. Proteomics 9, 3268–3284. doi: 10.1002/pmic.200800449
Jordy, M.-N. (2004). Seasonal variation of organogenetic activity and reserves allocation in the shoot apex of pinus pinaster ait. Ann. Bot. 93, 25–37. doi: 10.1093/aob/mch005
Kaup, M. T., Froese, C. D., Thompson, J. E. (2002). A role for diacylglycerol acyltransferase during leaf senescence. Plant Physiol. 129, 1616–1626. doi: 10.1104/pp.003087
Kelly, A. A., Quettier, A.-L., Shaw, E., Eastmond, P. J. (2011). Seed storage oil mobilization is important but not essential for germination or seedling establishment in arabidopsis. Plant Physiol. 157, 866–875. doi: 10.1104/pp.111.181784
Kelly, A. A., van Erp, H., Quettier, A.-L., Shaw, E., Menard, G., Kurup, S., et al. (2013). The sugar-dependent1 lipase limits triacylglycerol accumulation in vegetative tissues of arabidopsis. Plant Physiol. 162, 1282–1289. doi: 10.1104/pp.113.219840
Kilwein, M. D., Welte, M. A. (2019). Lipid droplet motility and organelle contacts. Contact Thousand Oaks Ventura Cty Calif 2. doi: 10.1177/2515256419895688
Kim, I., Kim, H. U. (2022). The mysterious role of fibrillin in plastid metabolism: current advances in understanding. J. Exp. Bot. 73, 2751–2764. doi: 10.1093/jxb/erac087
Kim, R. J., Kim, H. J., Shim, D., Suh, M. C. (2016). Molecular and biochemical characterizations of the monoacylglycerol lipase gene family of arabidopsis thaliana. Plant J. 85, 758–771. doi: 10.1111/tpj.13146
Kim, E. Y., Park, K. Y., Seo, Y. S., Kim, W. T. (2016). Arabidopsis small rubber particle protein homolog SRPs play dual roles as positive factors for tissue growth and development and in drought stress responses. Plant Physiol. 170, 2494–2510. doi: 10.1104/pp.16.00165
Kong, F., Romero, I. T., Warakanont, J., Li-Beisson, Y. (2018). Lipid catabolism in microalgae. New Phytol. 218, 1340–1348. doi: 10.1111/nph.15047
Korte, P., Unzner, A., Damm, T., Berger, S., Krischke, M., Mueller, M. J. (2023). High triacylglycerol turnover is required for efficient opening of stomata during heat stress in arabidopsis. Plant J. doi: 10.1111/tpj.16210
Krasensky, J., Jonak, C. (2012). Drought, salt, and temperature stress-induced metabolic rearrangements and regulatory networks. J. Exp. Bot. 63, 1593–1608. doi: 10.1093/jxb/err460
Krawczyk, H. E., Rotsch, A. H., Herrfurth, C., Scholz, P., Shomroni, O., Salinas-Riester, G., et al. (2022a). Heat stress leads to rapid lipid remodeling and transcriptional adaptations in Nicotiana tabacum pollen tubes. Plant Physiol. 189, 490–515. doi: 10.1093/plphys/kiac127
Krawczyk, H. E., Sun, S., Doner, N. M., Yan, Q., Lim, M. S. S., Scholz, P., et al. (2022b). Seed lipid droplet Protein1, seed lipid droplet Protein2, and lipid droplet plasma membrane adaptor mediate lipid droplet-plasma membrane tethering. Plant Cell 34, 2424–2448. doi: 10.1093/plcell/koac095
Kretzschmar, F. K., Doner, N. M., Krawczyk, H. E., Scholz, P., Schmitt, K., Valerius, O., et al. (2020). Identification of low-abundance lipid droplet proteins in seeds and seedlings. Plant Physiol. 182, 1326–1345. doi: 10.1104/pp.19.01255
Kretzschmar, F. K., Mengel, L. A., Müller, A. O., Schmitt, K., Blersch, K. F., Valerius, O., et al. (2018). PUX10 is a lipid droplet-localized scaffold protein that interacts with CELL DIVISION CYCLE48 and is involved in the degradation of lipid droplet proteins. Plant Cell 30, 2137–2160. doi: 10.1105/tpc.18.00276
Kurusu, T., Koyano, T., Hanamata, S., Kubo, T., Noguchi, Y., Yagi, C., et al. (2014). OsATG7 is required for autophagy-dependent lipid metabolism in rice postmeiotic anther development. Autophagy 10, 878–888. doi: 10.4161/auto.28279
Laibach, N., Schmidl, S., Müller, B., Bergmann, M., Prüfer, D., Gronover, C. S. (2018). Small rubber particle proteins from taraxacum brevicorniculatum promote stress tolerance and influence the size and distribution of lipid droplets and artificial poly(cis-1,4-isoprene) bodies. Plant J. 93, 1045–1061. doi: 10.1111/tpj.13829
Lass, A., Zimmermann, R., Haemmerle, G., Riederer, M., Schoiswohl, G., Schweiger, M., et al. (2006). Adipose triglyceride lipase-mediated lipolysis of cellular fat stores is activated by CGI-58 and defective in chanarin-dorfman syndrome. Cell Metab. 3, 309–319. doi: 10.1016/j.cmet.2006.03.005
Laufman, O., Perrino, J., Andino, R. (2019). Viral generated inter-organelle contacts redirect lipid flux for genome replication. Cell 178, 275–289.e16. doi: 10.1016/j.cell.2019.05.030
Lee, J., Yamaoka, Y., Kong, F., Cagnon, C., Beyly-Adriano, A., Jang, S., et al. (2020). The phosphatidylethanolamine-binding protein DTH1 mediates degradation of lipid droplets in chlamydomonas reinhardtii. Proc. Natl. Acad. Sci. 117, 23131–23139. doi: 10.1073/pnas.2005600117
Lersten, N. R., Czlapinski, A. R., Curtis, J. D., Freckmann, R., Horner, H. T. (2006). Oil bodies in leaf mesophyll cells of angiosperms: overview and a selected survey. Am. J. Bot. 93, 1731–1739. doi: 10.3732/ajb.93.12.1731
Li, F., Asami, T., Wu, X., Tsang, E. W. T., Cutler, A. J. (2007). A putative hydroxysteroid dehydrogenase involved in regulating plant growth and development. Plant Physiol. 145, 87–97. doi: 10.1104/pp.107.100560
Li, Y., Beisson, F., Pollard, M., Ohlrogge, J. (2006). Oil content of arabidopsis seeds: the influence of seed anatomy, light and plant-to-plant variation. Phytochemistry 67, 904–915. doi: 10.1016/j.phytochem.2006.02.015
Li, F., Han, X., Guan, H., Xu, M. C., Dong, Y. X., Gao, X.-Q. (2022). PALD encoding a lipid droplet-associated protein is critical for the accumulation of lipid droplets and pollen longevity in arabidopsis. New Phytol. 235, 204–219. doi: 10.1111/nph.18123
Li, Q., Zheng, Q., Shen, W., Cram, D., Fowler, D. B., Wei, Y., et al. (2015). Understanding the biochemical basis of temperature-induced lipid pathway adjustments in plants. Plant Cell 27, 86–103. doi: 10.1105/tpc.114.134338%JThePlantCell
Lin, L.-J., Tai, S. S. K., Peng, C.-C., Tzen, J. T. C. (2002). Steroleosin, a sterol-binding dehydrogenase in seed oil bodies. Plant Physiol. 128, 1200–1211. doi: 10.1104/pp.010982
Linssen, J. P. H., Cozijnsen, J. L., Pilnik, W. (1989). Chufa (cyperus esculentus): a new source of dietary fibre. J. Sci. Food Agric. 49, 291–296. doi: 10.1002/jsfa.2740490305
Liu, X., Yang, Z., Wang, Y., Shen, Y., Jia, Q., Zhao, C., et al. (2022). Multiple caleosins have overlapping functions in oil accumulation and embryo development. J. Exp. Bot. 73, 3946–3962. doi: 10.1093/jxb/erac153%JJournalofExperimentalBotany
Lopez, C., Sotin, H., Rabesona, H., Novales, B., Le Quéré, J.-M., Froissard, M., et al. (2023). Oil bodies from chia (Salvia hispanica l.) and camelina (Camelina sativa l.) seeds for innovative food applications: microstructure, composition and physical stability. Foods Basel Switz 12, 211. doi: 10.3390/foods12010211
Lundquist, P. K., Poliakov, A., Bhuiyan, N. H., Zybailov, B., Sun, Q., van Wijk, K. J. (2012). The functional network of the arabidopsis plastoglobule proteome based on quantitative proteomics and genome-wide coexpression analysis. Plant Physiol. 158, 1172–1192. doi: 10.1104/pp.111.193144
Lundquist, P. K., Shivaiah, K.-K., Espinoza-Corral, R. (2020). Lipid droplets throughout the evolutionary tree. Prog. Lipid Res. 78, 101029. doi: 10.1016/j.plipres.2020.101029
Marshall, R. S., Hua, Z., Mali, S., McLoughlin, F., Vierstra, R. D. (2019). ATG8-Binding UIM proteins define a new class of autophagy adaptors and receptors. Cell 177, 766–781.e24. doi: 10.1016/j.cell.2019.02.009
Masclaux-Daubresse, C., d’Andrea, S., Bouchez, I., Cacas, J.-L. (2020). Reserve lipids and plant autophagy. J. Exp. Bot. 71, 2854–2861. doi: 10.1093/jxb/eraa082
McLachlan, D. H., Lan, J., Geilfus, C.-M., Dodd, A. N., Larson, T., Baker, A., et al. (2016). The breakdown of stored triacylglycerols is required during light-induced stomatal opening. Curr. Biol. 26, 707–712. doi: 10.1016/j.cub.2016.01.019
Medina-Puche, L. (2019). Tailoring the cell: a glimpse of how plant viruses manipulate their hosts. Curr. Opin. Plant Biol. 52, 164–173. doi: 10.1016/j.pbi.2019.09.007
Mellema, S., Eichenberger, W., Rawyler, A., Suter, M., Tadege, M., Kuhlemeier, C. (2002). The ethanolic fermentation pathway supports respiration and lipid biosynthesis in tobacco pollen. Plant J. 30, 329–336. doi: 10.1046/j.1365-313X.2002.01293.x
Michaud, M., Jouhet, J. (2019). Lipid trafficking at membrane contact sites during plant development and stress response. Front. Plant Sci. 10. doi: 10.3389/fpls.2019.00002
Michel, E. J. S., Ponnala, L., van Wijk, K. J. (2021). Tissue-type specific accumulation of the plastoglobular proteome, transcriptional networks, and plastoglobular functions. J. Exp. Bot. 72, 4663–4679. doi: 10.1093/jxb/erab175
Miklaszewska, M., Zienkiewicz, K., Klugier-Borowska, E., Rygielski, M., Feussner, I., Zienkiewicz, A. (2023). Caleosin 1 contributes to seed lipid droplet degradation by interaction with autophagy-related protein ATG8. bioRxiv 2023.04.04.535563. doi: 10.1101/2023.04.04.535563
Miquel, M., Trigui, G., d’Andréa, S., Kelemen, Z., Baud, S., Berger, A., et al. (2014). Specialization of oleosins in oil body dynamics during seed development in arabidopsis seeds. Plant Physiol. 164, 1866–1878. doi: 10.1104/pp.113.233262
Miray, R., Kazaz, S., To, A., Baud, S. (2021). Molecular control of oil metabolism in the endosperm of seeds. Int. J. Mol. Sci. 22, 1621. doi: 10.3390/ijms22041621
Miwa, T. K. (1971). Jojoba oil wax esters and derived fatty acids and alcohols: gas chromatographic analyses. J. Am. Oil Chem. Soc 48, 259–264. doi: 10.1007/BF02638458
Moellering, E. R., Benning, C. (2010). RNA Interference silencing of a major lipid droplet protein affects lipid droplet size in chlamydomonas reinhardtii. Eukaryot Cell 9, 97–106. doi: 10.1128/EC.00203-09
Moellering, E. R., Muthan, B., Benning, C. (2010). Freezing tolerance in plants requires lipid remodeling at the outer chloroplast membrane. Science 330, 226–228. doi: 10.1126/science.1191803
Mueller, S. P., Krause, D. M., Mueller, M. J., Fekete, A. (2015). Accumulation of extra-chloroplastic triacylglycerols in arabidopsis seedlings during heat acclimation. J. Exp. Bot. 66, 4517–4526. doi: 10.1093/jxb/erv226
Mueller, S. P., Unger, M., Guender, L., Fekete, A., Mueller, M. J. (2017). Phospholipid : diacylglycerol acyltransferase-mediated triacylglyerol synthesis augments basal thermotolerance. Plant Physiol. 175, 486–497. doi: 10.1104/pp.17.00861
Müller, A. O., Ischebeck, T. (2018). Characterization of the enzymatic activity and physiological function of the lipid droplet-associated triacylglycerol lipase AtOBL1. New Phytol. 217, 1062–1076. doi: 10.1111/nph.14902
Murphy, D. (2001). The biogenesis and functions of lipid bodies in animals, plants and microorganisms. Prog. Lipid Res. 40, 325–438. doi: 10.1016/S0163-7827(01)00013-3
Nagy, P., Pogany, J., Xu, K. (2016). Cell-free and cell-based approaches to explore the roles of host membranes and lipids in the formation of viral replication compartment induced by tombusviruses. Viruses 8, 68. doi: 10.3390/v8030068
Narayanan, S., Tamura, P. J., Roth, M. R., Prasad, P. V. V., Welti, R. (2016). Wheat leaf lipids during heat stress: i. high day and night temperatures result in major lipid alterations. Plant Cell Environ. 39, 787–803. doi: 10.1111/pce.12649
Nguyen, H. M., Baudet, M., Cuiné, S., Adriano, J.-M., Barthe, D., Billon, E., et al. (2011). Proteomic profiling of oil bodies isolated from the unicellular green microalga chlamydomonas reinhardtii: with focus on proteins involved in lipid metabolism. PROTEOMICS 11, 4266–4273. doi: 10.1002/pmic.201100114
Niemeyer, P. W., Irisarri, I., Scholz, P., Schmitt, K., Valerius, O., Braus, G. H., et al. (2022). A seed-like proteome in oil-rich tubers. Plant J. 112, 518–534. doi: 10.1111/tpj.15964
Oh, S. K., Kang, H., Shin, D. H., Yang, J., Chow, K.-S., Yeang, H. Y., et al. (1999). Isolation, characterization, and functional analysis of a novel cDNA clone encoding a small rubber particle protein from hevea brasiliensis *. J. Biol. Chem. 274, 17132–17138. doi: 10.1074/jbc.274.24.17132
Park, S., Gidda, S. K., James, C. N., Horn, P. J., Khuu, N., Seay, D. C., et al. (2013). The α/β hydrolase CGI-58 and peroxisomal transport protein PXA1 coregulate lipid homeostasis and signaling in arabidopsis. Plant Cell 25, 1726–1739. doi: 10.1105/tpc.113.111898
Pautov, A., Koteyeva, N., Yakovleva, O., Ivanova, A., Krylova, E., Tarasova, M., et al. (2022). Large Lipid droplets of the guard cells are dynamic organelles of the functioning stomata of fagraea ceilanica. Flora 297, 152182. doi: 10.1016/j.flora.2022.152182
Pautov, A., Yakovleva, O., Krylova, E., Gussarova, G. (2016). Large Lipid droplets in leaf epidermis of angiosperms. Flora 219, 62–67. doi: 10.1016/j.flora.2015.12.010
Pautov, A., Yakovleva, O., Krylova, E., Pautova, I., Gussarova, G. (2018). Structural changes of large lipid droplets in stomatal complex of trochodendron aralioides and their possible functional significance. Flora 242, 146–154. doi: 10.1016/j.flora.2018.03.016
Penfield, S., Rylott, E. L., Gilday, A. D., Graham, S., Larson, T. R., Graham, I. A. (2004). Reserve mobilization in the arabidopsis endosperm fuels hypocotyl elongation in the dark, is independent of abscisic acid, and requires PHOSPHOENOLPYRUVATE CARBOXYKINASE1. Plant Cell 16, 2705–2718. doi: 10.1105/tpc.104.024711
Piffanelli, P., Ross, J. H. E., Murphy, D. J. (1997). Intra- and extracellular lipid composition and associated gene expression patterns during pollen development in brassica napus. Plant J. 11, 549–562. doi: 10.1046/j.1365-313X.1997.11030549.x
Piffanelli, P., Ross, J. H. E., Murphy, D. J. (1998). Biogenesis and function of the lipidic structures of pollen grains. Sex Plant Reprod. 11, 65–80. doi: 10.1007/s004970050122
Pospisil, P. (2016). Production of reactive oxygen species by photosystem II as a response to light and temperature stress. Front. Plant Sci. 7. doi: 10.3389/fpls.2016.01950
Poxleitner, M., Rogers, S. W., Lacey Samuels, A., Browse, J., Rogers, J. C. (2006). A role for caleosin in degradation of oil-body storage lipid during seed germination. Plant J. 47, 917–933. doi: 10.1111/j.1365-313X.2006.02845.x
Pyc, M., Gidda, S. K., Seay, D., Esnay, N., Kretzschmar, F. K., Cai, Y., et al. (2021). LDIP cooperates with SEIPIN and LDAP to facilitate lipid droplet biogenesis in arabidopsis. Plant Cell 33, 3076–3103. doi: 10.1093/plcell/koab179
Quettier, A.-L., Eastmond, P. J. (2009). Storage oil hydrolysis during early seedling growth. Plant Physiol. Biochem. PPB 47, 485–490. doi: 10.1016/j.plaphy.2008.12.005
Rajangam, A. S., Gidda, S. K., Craddock, C., Mullen, R. T., Dyer, J. M., Eastmond, P. J. (2013). Molecular characterization of the fatty alcohol oxidation pathway for wax-ester mobilization in germinated jojoba Seeds1[W]. Plant Physiol. 161, 72–80. doi: 10.1104/pp.112.208264
Rinne, P. L. H., Kaikuranta, P. L. M., van der Plas, L. H. W., van der Schoot, C. (1999). Dehydrins in cold-acclimated apices of birch (Betula pubescens ehrh.): production, localization and potential role in rescuing enzyme function during dehydration. Planta 209, 377–388. doi: 10.1007/s004250050740
Rinne, P. L., Kaikuranta, P. M., van der Schoot, C. (2001). The shoot apical meristem restores its symplasmic organization during chilling-induced release from dormancy. Plant J. Cell Mol. Biol. 26, 249–264. doi: 10.1046/j.1365-313x.2001.01022.x
Rinne, P. L. H., Welling, A., Vahala, J., Ripel, L., Ruonala, R., Kangasjärvi, J., et al. (2011). Chilling of dormant buds hyperinduces FLOWERING LOCUS T and recruits GA-inducible 1,3-β-Glucanases to reopen signal conduits and release dormancy in populus. Plant Cell 23, 130–146. doi: 10.1105/tpc.110.081307
Rodríguez-García, M. I., M’rani-Alaoui, M., Fernández, M. C. (2003). Behavior of storage lipids during development and germination of olive (Olea europaea l.) pollen. Protoplasma 221, 237–244. doi: 10.1007/s00709-002-0076-x
Roingeard, P., Melo, R. C. N. (2017). Lipid droplet hijacking by intracellular pathogens. Cell. Microbiol. 19, e12688. doi: 10.1111/cmi.12688
Rost, T. L., Paterson, K. E. (1978). Structural and histochemical characterization of the cotyledon storage organelles of jojoba (Simmondsia chinensis). Protoplasma 95, 1–10. doi: 10.1007/BF01279690
Rotsch, A. H., Kopka, J., Feussner, I., Ischebeck, T. (2017). Central metabolite and sterol profiling divides tobacco male gametophyte development and pollen tube growth into eight metabolic phases. Plant J. 92, 129–146. doi: 10.1111/tpj.13633
Sagisaka, S. (1992). A cold environment is a prerequisite for formation of “Plastid initials” in winter buds of poplar. Plant Physiol. 99, 1657–1663. doi: 10.1104/pp.99.4.1657
Schieferle, S., Tappe, B., Korte, P., Mueller, M. J., Berger, S. (2021). Pathogens and elicitors induce local and systemic changes in triacylglycerol metabolism in roots and in leaves of arabidopsis thaliana. Biology 10, 920. doi: 10.3390/biology10090920
Scholz, P., Chapman, K. D., Mullen, R. T., Ischebeck, T. (2022). Finding new friends and revisiting old ones – how plant lipid droplets connect with other subcellular structures. New Phytol. 236, 833–838. doi: 10.1111/nph.18390
Sham, A., Al-Azzawi, A., Al-Ameri, S., Al-Mahmoud, B., Awwad, F., Al-Rawashdeh, A., et al. (2014). Transcriptome analysis reveals genes commonly induced by botrytis cinerea infection, cold, drought and oxidative stresses in arabidopsis. PloS One 9, e113718. doi: 10.1371/journal.pone.0113718
Shimada, T. L., Takano, Y., Shimada, T., Fujiwara, M., Fukao, Y., Mori, M., et al. (2014). Leaf oil body functions as a subcellular factory for the production of a phytoalexin in arabidopsis. Plant Physiol. 164, 105–118. doi: 10.1104/pp.113.230185
Shiva, S., Samarakoon, T., Lowe, K. A., Roach, C., Vu, H. S., Colter, M., et al. (2020). Leaf lipid alterations in response to heat stress of arabidopsis thaliana. Plants Basel Switz 9, 845. doi: 10.3390/plants9070845
Siloto, R. M. P., Findlay, K., Lopez-Villalobos, A., Yeung, E. C., Nykiforuk, C. L., Moloney, M. M. (2006). The accumulation of oleosins determines the size of seed oilbodies in arabidopsis. Plant Cell 18, 1961–1974. doi: 10.1105/tpc.106.041269
Singh, R., Kaushik, S., Wang, Y., Xiang, Y., Novak, I., Komatsu, M., et al. (2009). Autophagy regulates lipid metabolism. Nature 458, 1131–1135. doi: 10.1038/nature07976
Slocombe, S. P., Cornah, J., Pinfield-Wells, H., Soady, K., Zhang, Q., Gilday, A., et al. (2009). Oil accumulation in leaves directed by modification of fatty acid breakdown and lipid synthesis pathways. Plant Biotechnol. J. 7, 694–703. doi: 10.1111/j.1467-7652.2009.00435.x
Sturtevant, D., Dueñas, M. E., Lee, Y. J., Chapman, K. D. (2017). Three-dimensional visualization of membrane phospholipid distributions in arabidopsis thaliana seeds: a spatial perspective of molecular heterogeneity. Biochim. Biophys. Acta Mol. Cell Biol. Lipids 1862, 268–281. doi: 10.1016/j.bbalip.2016.11.012
Sturtevant, D., Lu, S., Zhou, Z. W., Shen, Y., Wang, S., Song, J. M., et al. (2020). The genome of jojoba (Simmondsia chinensis): a taxonomically isolated species that directs wax ester accumulation in its seeds. Sci. Adv. 6, 3240. doi: 10.1126/sciadv.aay3240
Sun, A.-Z., Chen, L.-S., Tang, M., Chen, J.-H., Li, H., Jin, X.-Q., et al. (2022). Lipidomic remodeling in begonia grandis under heat stress. Front. Plant Sci. 13. doi: 10.3389/fpls.2022.843942
Sutinen, S., Partanen, J., Viherä-Aarnio, A., Häkkinen, R. (2009). Anatomy and morphology in developing vegetative buds on detached Norway spruce branches in controlled conditions before bud burst. Tree Physiol. 29, 1457–1465. doi: 10.1093/treephys/tpp078
Sutinen, S., Partanen, J., Viherä-Aarnio, A., Häkkinen, R. (2012). Development and growth of primordial shoots in Norway spruce buds before visible bud burst in relation to time and temperature in the field. Tree Physiol. 32, 987–997. doi: 10.1093/treephys/tps063
Tan, W. J., Yang, Y. C., Zhou, Y., Huang, L. P., Xu, L., Chen, Q. F., et al. (2018). Diacylglycerol acyltransferase and diacylglycerol kinase modulate triacylglycerol and phosphatidic acid production in the plant response to freezing stress. Plant Physiol. 177, 1303–1318. doi: 10.1104/pp.18.00402
Taurino, M., Costantini, S., De Domenico, S., Stefanelli, F., Ruano, G., Delgadillo, M. O., et al. (2018). SEIPIN proteins mediate lipid droplet biogenesis to promote pollen transmission and reduce seed dormancy. Plant Physiol. 176, 1531–1546. doi: 10.1104/pp.17.01430
Thazar-Poulot, N., Miquel, M., Fobis-Loisy, I., Gaude, T. (2015). Peroxisome extensions deliver the arabidopsis SDP1 lipase to oil bodies. Proc. Natl. Acad. Sci. 112, 4158–4163. doi: 10.1073/pnas.1403322112
Troncoso-Ponce, M. A., Cao, X., Yang, Z., Ohlrogge, J. B. (2013). Lipid turnover during senescence. Plant Sci. 205–206, 13–19. doi: 10.1016/j.plantsci.2013.01.004
Turesson, H., Marttila, S., Gustavsson, K.-E., Hofvander, P., Olsson, M. E., Bülow, L., et al. (2010). Characterization of oil and starch accumulation in tubers of cyperus esculentus var. sativus (Cyperaceae): a novel model system to study oil reserves in nonseed tissues. Am. J. Bot. 97, 1884–1893. doi: 10.3732/ajb.1000200
Uemura, M., Joseph, R. A., Steponkus, P. L. (1995). Cold acclimation of arabidopsis thaliana (Effect on plasma membrane lipid composition and freeze-induced lesions). Plant Physiol. 109, 15–30. doi: 10.1104/pp.109.1.15%JPlantPhysiology
van Wijk, K. J., Kessler, F. (2017). Plastoglobuli: plastid microcompartments with integrated functions in metabolism, plastid developmental transitions, and environmental adaptation. Annu. Rev. Plant Biol. 68, 253–289. doi: 10.1146/annurev-arplant-043015-111737
Veerabagu, M., Paul, L. K., Rinne, P. L., van der Schoot, C. (2020). Plant lipid bodies traffic on actin to plasmodesmata motorized by myosin XIs. Int. J. Mol. Sci. 21, 1422. doi: 10.3390/ijms21041422
Veerabagu, M., Rinne, P. L. H., Skaugen, M., Paul, L. K., van der Schoot, C. (2021). Lipid body dynamics in shoot meristems: production, enlargement, and putative organellar interactions and plasmodesmal targeting. Front. Plant Sci. 12. doi: 10.3389/fpls.2021.674031
Vidi, P.-A., Kanwischer, M., Baginsky, S., Austin, J. R., Csucs, G., Dörmann, P., et al. (2006). Tocopherol cyclase (VTE1) localization and vitamin e accumulation in chloroplast plastoglobule lipoprotein particles *. J. Biol. Chem. 281, 11225–11234. doi: 10.1074/jbc.M511939200
Vieler, A., Brubaker, S. B., Vick, B., Benning, C. (2012). A lipid droplet protein of nannochloropsis with functions partially analogous to plant oleosins. Plant Physiol. 158, 1562–1569. doi: 10.1104/pp.111.193029
Wang, H., Becuwe, M., Housden, B. E., Chitraju, C., Porras, A. J., Graham, M. M., et al. (2016). Seipin is required for converting nascent to mature lipid droplets. eLife 5, e16582. doi: 10.7554/eLife.16582
Welte, M. A., Gould, A. P. (2017). Lipid droplet functions beyond energy storage. Biochim. Biophys. Acta Mol. Cell Biol. Lipids 1862, 1260–1272. doi: 10.1016/j.bbalip.2017.07.006
Woodfield, H. K., Sturtevant, D., Borisjuk, L., Munz, E., Guschina, I. A., Chapman, K., et al. (2017). Spatial and temporal mapping of key lipid species in brassica napus seeds. Plant Physiol. 173, 1998–2009. doi: 10.1104/pp.16.01705
Xu, H., Cao, D., Chen, Y., Wei, D., Wang, Y., Stevenson, R. A., et al. (2016). Gene expression and proteomic analysis of shoot apical meristem transition from dormancy to activation in cunninghamia lanceolata (Lamb.) hook. Sci. Rep. 6, 19938. doi: 10.1038/srep19938
Xu, C., Shanklin, J. (2016). Triacylglycerol metabolism, function, and accumulation in plant vegetative tissues. Annu. Rev. Plant Biol. 67, 179–206. doi: 10.1146/annurev-arplant-043015-111641
Yang, L.-N., Liu, H., Wang, Y.-P., Seematti, J., Grenville-Briggs, L. J., Wang, Z., et al. (2021). Pathogen-mediated stomatal opening: a previously overlooked pathogenicity strategy in the oomycete pathogen phytophthora infestans. Front. Plant Sci. 12. doi: 10.3389/fpls.2021.668797
Yang, Z., Ohlrogge, J. B. (2009). Turnover of fatty acids during natural senescence of arabidopsis, brachypodium, and switchgrass and in arabidopsis β-oxidation mutants. Plant Physiol. 150, 1981–1989. doi: 10.1104/pp.109.140491
Yoshimoto, K., Ohsumi, Y. (2018). Unveiling the molecular mechanisms of plant autophagy-from autophagosomes to vacuoles in plants. Plant Cell Physiol. 59, 1337–1344. doi: 10.1093/pcp/pcy112
You, Z., Zhang, Q., Peng, Z., Miao, X. (2019). Lipid droplets mediate salt stress tolerance in Parachlorella kessleri 1. Plant Physiol. 181, 510–526. doi: 10.1104/pp.19.00666
Yurchenko, O., Kimberlin, A., Mehling, M., Koo, A. J., Chapman, K. D., Mullen, R. T., et al. (2018). Response of high leaf-oil Arabidopsis thaliana plant lines to biotic or abiotic stress. Plant Signal. Behav. 13, e1464361. doi: 10.1080/15592324.2018.1464361
Zaaboul, F., Raza, H., Chen, C., Liu, Y. (2018). Characterization of peanut oil bodies integral proteins, lipids, and their associated phytochemicals. J. Food Sci. 83, 93–100. doi: 10.1111/1750-3841.13995
Zhang, Z., Cheng, Z., Gan, L., Zhang, H., Wu, F., Lin, Q., et al. (2016). OsHSD1, a hydroxysteroid dehydrogenase, is involved in cuticle formation and lipid homeostasis in rice. Plant Sci. 249, 35–45. doi: 10.1016/j.plantsci.2016.05.005
Zhang, M., Fan, J., Taylor, D. C., Ohlrogge, J. B. (2009). DGAT1 and PDAT1 acyltransferases have overlapping functions in arabidopsis triacylglycerol biosynthesis and are essential for normal pollen and seed development. Plant Cell 21, 3885–3901. doi: 10.1105/tpc.109.071795
Zhang, Z., He, G., Filipowicz, N. A., Randall, G., Belov, G. A., Kopek, B. G., et al. (2019). Host lipids in positive-strand RNA virus genome replication. Front. Microbiol. 10. doi: 10.3389/fmicb.2019.00286
Zhang, Y., Li, Y., Han, B., Liu, A., Xu, W. (2022). Integrated lipidomic and transcriptomic analysis reveals triacylglycerol accumulation in castor bean seedlings under heat stress. Ind. Crops Prod. 180, 114702. doi: 10.1016/j.indcrop.2022.114702
Zhang, C., Qu, Y., Lian, Y., Chapman, M., Chapman, N., Xin, J., et al. (2020). A new insight into the mechanism for cytosolic lipid droplet degradation in senescent leaves. Physiol. Plant 168, 835–844. doi: 10.1111/ppl.13039
Zhao, L., Dai, J., Wu, Q. (2014). Autophagy-like processes are involved in lipid droplet degradation in auxenochlorella protothecoides during the heterotrophy-autotrophy transition. Front. Plant Sci. 5. doi: 10.3389/fpls.2014.00400
Zhao, P., Zhou, X.-M., Zhao, L.-L., Cheung, A. Y., Sun, M.-X. (2020). Autophagy-mediated compartmental cytoplasmic deletion is essential for tobacco pollen germination and male fertility. Autophagy 16, 2180–2192. doi: 10.1080/15548627.2020.1719722
Zheng, Y., Deng, X., Qu, A., Zhang, M., Tao, Y., Yang, L., et al. (2018). Regulation of pollen lipid body biogenesis by MAP kinases and downstream WRKY transcription factors in arabidopsis. PloS Genet. 14, e1007880. doi: 10.1371/journal.pgen.1007880
Zhi, Y., Taylor, M. C., Campbell, P. M., Warden, A. C., Shrestha, P., El Tahchy, A., et al. (2017). Comparative lipidomics and proteomics of lipid droplets in the mesocarp and seed tissues of Chinese tallow (Triadica sebifera). Front. Plant Sci. 8, 1339. doi: 10.3389/fpls.2017.01339
Zhou, X., Chen, X., Du, Z., Zhang, Y., Zhang, W., Kong, X., et al. (2019). Terpenoid esters are the major constituents from leaf lipid droplets of camellia sinensis. Front. Plant Sci. 10. doi: 10.3389/fpls.2019.00179
Zienkiewicz, K., Zienkiewicz, A. (2020). Degradation of lipid droplets in plants and algae–right time, many paths, one goal. Front. Plant Sci. 11. doi: 10.3389/fpls.2020.579019
Zienkiewicz, A., Zienkiewicz, K., Rejón, J. D., Rodríguez-García, M. I., Castro, A. J. (2013). New insights into the early steps of oil body mobilization during pollen germination. J. Exp. Bot. 64, 293–302. doi: 10.1093/jxb/ers332
Zita, W., Bressoud, S., Glauser, G., Kessler, F., Shanmugabalaji, V. (2022). Chromoplast plastoglobules recruit the carotenoid biosynthetic pathway and contribute to carotenoid accumulation during tomato fruit maturation. PloS One 17, e0277774. doi: 10.1371/journal.pone.0277774
Zoeller, M., Stingl, N., Krischke, M., Fekete, A., Waller, F., Berger, S., et al. (2012). Lipid profiling of the arabidopsis hypersensitive response reveals specific lipid peroxidation and fragmentation processes: biogenesis of pimelic and azelaic acid. Plant Physiol. 160, 365–378. doi: 10.1104/pp.112.202846
Keywords: lipid droplets, environmental stress, triacylglycerol, membrane remodeling, autophagy, lipolysis, heat, post-stress recovery
Citation: Bouchnak I, Coulon D, Salis V, D’Andréa S and Bréhélin C (2023) Lipid droplets are versatile organelles involved in plant development and plant response to environmental changes. Front. Plant Sci. 14:1193905. doi: 10.3389/fpls.2023.1193905
Received: 25 March 2023; Accepted: 23 May 2023;
Published: 23 June 2023.
Edited by:
Eric Marechal, UMR5168 Laboratoire de Physiologie Cellulaire Vegetale (LPCV), FranceReviewed by:
Till Ischebeck, University of Münster, GermanyMartin Potocký, Academy of Sciences of the Czech Republic, Czechia
Copyright © 2023 Bouchnak, Coulon, Salis, D’Andréa and Bréhélin. This is an open-access article distributed under the terms of the Creative Commons Attribution License (CC BY). The use, distribution or reproduction in other forums is permitted, provided the original author(s) and the copyright owner(s) are credited and that the original publication in this journal is cited, in accordance with accepted academic practice. No use, distribution or reproduction is permitted which does not comply with these terms.
*Correspondence: Claire Bréhélin, claire.brehelin@u-bordeaux.fr