Abstract
Insect galls are abnormal plant organs formed by gall-inducing insects to provide shelter and nutrients for themselves. Although insect galls are spatialized complex structures with unique shapes and functions, the molecular mechanism of the gall formation and the screening system for the gall inducing effectors remains unknown. Here, we demonstrate that an extract of a gall-inducing aphid, Schlechtendalia chinensis, induces an abnormal structure in the root-tip region of Arabidopsis seedlings. The abnormal structure is composed of stem-like cells, vascular, and protective tissues, as observed in typical insect galls. Furthermore, we confirm similarities in the gene expression profiles between the aphid-treated seedlings and the early developmental stages of Rhus javanica galls formed by S. chinensis. Based on the results, we propose a model system for analyzing the molecular mechanisms of gall formation: the Arabidopsis-based Gall-Forming Assay (Ab-GALFA). Ab-GALFA could be used not only as a model to elucidate the mechanisms underlying gall formation, but also as a bioassay system to isolate insect effector molecules of gall-induction.
Similar content being viewed by others
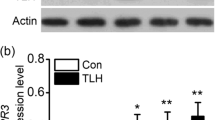
Introduction
Galls on plants are abnormal organs formed by various plant parasites including viruses, fungi, bacteria, nematodes, and insects. Insect galls have extremely complex and highly organized structures comprising several specialized tissues that the insects utilize for shelter and nutrients1,2. Many remarkable flower- and fruit-like structures have been observed in insect galls, particularly those induced by gall midges, aphids, and cynipids in various host plant species3, suggesting that the formation of gall tissues is similar to that of flowers or fruits4,5.
We have previously performed RNA-sequencing-based comparative transcriptomic analysis of the early developmental stage of horned gall to elucidate the early gall-inducing process carried out by a gall-inducing aphid, Schlechtendalia chinensis, in the Chinese sumac, Rhus javanica, and found that several reproductive organ genes are expressed in the early development phase of galls induced6. Furthermore, the comparative study of three-different galls induce on Artemisia montana, Glochidion obovatum, and R. javanica indicated that several key regulators of stem cell generation (CLE44, BAM3, WOXs) and maintenance floral organ development (SEPALLATA, AGAMOUS, and APETALA1) were commonly upregulated during gall development7,8.
In the plant tissues level, insect galls include (i) callus-like cells transformed from palisade and spongy tissues used for insect feeding in inner-layer cells (ii) protective outer tissue composed of lignified secondary cell walls for shelter and protection in outer-layer cells, and (iii) vascular tissues which transports water and nutrients to the callus-like cells in the gall9,10,11.
To generate these complex gall structures, gall-inducing insects are believed to manipulate plant developmental programs by secreting effector molecules2,12,13. In fact, phytohormones, including significant quantities of auxins and cytokinins, as well as certain amino acids and proteins, have been detected in gall-inducing insects2,14,15,16,17,18. Such chemical stimuli are produced via the salivary secretions and oviposition fluids produced by larvae, parent insects, or both13,14,15.
Thus, gall-inducing insects secrete effector molecules into plant tissues using their mouthparts or ovipositors to manipulate plant development, thereby generating complex gall structures in host plants1,2,13. However, owing to the lack of model symbiotic systems for dissecting the molecular mechanisms of insect gall formation, the effector molecules and mechanisms underlying the gall formation process in plants remain largely unknown.
We hypothesize that the effectors control the developmental pathways in the model plant A. thaliana, as well as in the host plants, since the molecular machinery for development and the developmental pathways are highly conserved among higher plants.
Here, we develop the Arabidopsis-based Gall Formation Assay (Ab-GALFA), an effective method of analyzing the molecular mechanisms underlying gall formation using a model plant, Arabidopsis thaliana. This model system could be used to elucidate the molecular basis for gall formation in plants, and can be utilized as a bioassay for isolating the unknown insect effectors of gall formation in various host plants.
Results
Extract of a gall-inducing insect can alter the morphology of the model plant, A. thaliana
We determined whether treating the seedlings of the model plant, A. thaliana, with an extract of a gall-inducing insect would induce morphological changes in the Arabidopsis tissues. As a first attempt, we tested S. chinensis as a model gall-inducing insect for the following reasons: (i) S. chinensis induces large gall over a short period, (ii) a large volume of clone insect bodies could be available in one gall tissue, (iii) we have previously investigated the expression profile of the early stage of gall (Fig. 1A,B)6.
Firstly, morphological changes in 4-day-old Arabidopsis seedlings soaked in various concentrations of the extract of gall-inducing aphid, S. chinensis, (hereafter referred to as Sc extract) were observed via light microscopy after 1 day of treatment (Fig. 1C). We found that several morphologies of the seedlings were significantly changed. For example, the root and elongation zones abnormally thickened, with several thick root hair-like structures that emerged from the root elongation zone (Fig. 2A and B, Supplemental Movie 1). These morphological changes were not observed following treatment with extracts of the non-galling aphid species Acyrthosiphon pisum (A. p) and Megoura crassicauda (M. c) (Fig. S1–S5), suggesting that the phenomenon was caused specifically by the extract of the gall-inducing insect.
Schematic illustration explaining the use of Ab-GALFA for testing the gall-inducing effect of an aphid, Schlechtendalia chinensis. S. chinensis (A) induces large, single-chamber galls (B) in Rhus javanica. (C) Insect bodies are ground in liquid nitrogen and suspended in sterile distilled water to prepare the insect extract solution. Arabidopsis seedlings incubated in the solution are observed or analyzed via confocal microscopy or RNA sequencing.
Morphological changes in the root-tip region of Arabidopsis after S. chinensis extract treatment. The morphology of the root-tip region of Arabidopsis seedlings without (A) or with (B) S. chinensis extract treatment. Propidium iodide-stained root tips of Arabidopsis seedlings without (C) or with (D) S. chinensis extract treatment. The length (E) and width (F) of the root epidermal cells were measured from the initial root epidermal cell. Numbers represent the cell number from the initial cell. Blue: control, orange: after S. chinensis extract treatment. Asterisks denote significant differences based on the Mann–Whitney U-test (p < 0.05); n = 3 biological replicates of five seedlings each. Scale bars: 100 µm (A–D).
We then examined the morphological changes that occur at the cellular level. We stained Sc extract-treated Arabidopsis seedlings with propidium iodide (Fig. 2C and D) and measured the vertical and horizontal lengths of the epidermal cells of the root-tip region. The width of the root epidermal cells from the division zone to the transition zone was increased, whereas the length of the root epidermal cells was only increased in the transition zone, when the seedlings were incubated with Sc extract. (Fig. 2E and F). From these results, we concluded that the morphological changes in the root-tip region induced by the the Sc extract were attributed to changes in the wider shape of the root epidermal cells from the division to the transition zones in the root.
The morphological changes in the root epidermal cells following treatment with the Sc extract might have been caused by changes in the cytoskeleton and/or organelle structures. A cytoskeletal structure, cortical microtubules, and the most prominent plant organelle, the central vacuole, are important for the construction of cell shape in plants19,20. Therefore, we investigated the mechanism by which the morphology of the cortical microtubules and central vacuoles were altered by the Sc extract treatment. The parallel alignment of the cortical microtubule array visualized using a fluorescent microtubule marker, GFP-MBD21. Measurement of the microtubule density of root epidermal cells of root elongation zone indicated that the cortical microtubule array was severely disordered by the Sc extract treatment (Fig. 3A–C).
Changes in the structure of cortical microtubules and vacuoles in root epidermal cells after aphid extract treatment. (A–C) Live cell imaging of Arabidopsis root-transition zone expressing microtubule fluorescent markers GFP-MBD without (A) or with (B) S. chinensis extract treatment; (C) The density of cortical microtubules. The asterisk denotes a statistically significant difference between the control and Sc extract-treated conditions (*p < 0.05; Mann–Whitney U-test). Biological replicates of 18 seedlings. In the box-and-whisker plots, the boxes and solid lines in the boxes show the upper (75th) and lower (25th) quartiles and median values, respectively. The whiskers indicate the 95% confidence intervals. The experiments were independently repeated three times. (D–G) Live cell imaging of Arabidopsis root-transition zone and root division zone of transgenic Arabidopsis line expressing a vacuole membrane fluorescent marker GFP-AtVAM3 without (D, F) or with (E, G) S. chinensis extract treatment. Scale bars: 10 μm.
Plant vacuoles have a dynamic membrane system that undergoes complex architectural remodeling during developmental process22,23. In particular, dynamic morphological remodeling, in which fragmented small vacuoles are fused and form large central vacuolar structures, occurs during the development of mature root epidermal cells from root meristematic cells24,25. After treatment with the Sc extract, the bursiform and complex structures of the vacuoles in the root meristematic and elongation zones changed to more fragmented, smaller vacuolar structures (Fig. 3D–G) that resembled the vacuolar structures in root meristematic cells24.
S. chinensis extract contains significant amounts of phytohormones
Gall-inducing insects are known to produce phytohormones in their bodies and inject them into host plants to induce gall-structure development13,14,17,26,27,28. The application of appropriate combinations and concentrations of exogenous phytohormones can induce gall-like structures in plants28. These observations imply that the gall-like structure that emerged in the root region following treatment with the the Sc extract might be caused by exogenous phytohormones from the aphid bodies. Therefore, we measured the concentrations of typical phytohormones in S. chinensis and considerable quantities of abscisic acid (ABA), salicylic acid (SA), jasmonic acid (JA), jasmonoyl-L-isoleucine (JA-Ile) were detected in addition to the presence of indole acetic acid (IAA), isopentyladenine (iP), and trans-zeatin (tZ) (Table 1), suggesting that various types of phytohormones can function as insect effector molecules for gall-induction.
Typical characteristics of insect gall-like structures that emerged in the root-tip region after the aphid extract and phytohormone mixture treatments
Typical insect galls consist of three representative tissues: stem cells, vascular, and protective tissues1,2. We tested whether these three representative gall tissues (stem cells vascular, and protective tissues) could be observed in the gall-like structures in the root region of the Arabidopsis seedlings after the treatment with the Sc extract or an artificial phytohormone mixture (AHM), which mimicked the content and concentration of the phytohormones in the Sc extract.
As previously reported, several key regulatory molecules in stem cell generation are expressed during the initial stages of gall formation5,6,7. Therefore, we used a stem cell niche marker, PLETHORA1 (PLT1)29, and a quiescent center (QC) marker, WUSCHEL RELETATED HOMEOBOX (WOX5)30 to determine whether the structure of the stem cell niche was altered following treatment with the Sc extract or AHM. PLT1-GFP fluorescence was also observed not only in the epidermal cells of the division zone but also of the root transition, elongation and division zones in the Sc extract treatment (Fig. 4). The ectopic expression of PLT1 in the cells of the root-transition and elongation zone was not observed following treatment with the extracts of A. pisum and M. crassicauda, AHM, and a control treatment (water) (Fig. 4 and Fig. S1). A QC marker, WOX5p::WOX5-GUS-mNeonGreen exhibited similar expression pattern of the PLT1p::PLT1-GFP in root-tip region (Fig. S2).
S. chinensis extract initialized epidermal cells of root elongation zone. (A) Fluorescence imaging of PLT1-GFP expressing lines without or with treatment using an artificial hormone mix (AHM) solution or S. chinensis extract. Scale bars = 100 µm. (B) Box-and-whisker plots showing the fluorescence intensity in (A) (n = 8 seedlings for each treatment, with three biological replicates). The boxes and solid lines in the boxes show the upper (75th) and lower (25th) quartiles and median values, respectively. Groups with different letters are significantly different from each other (p < 0.05, Wilcoxon and Steel–Dwass tests).
These results indicated that the stem cell niche spread from the root-meristematic region to the root- elongation zones only after the Sc extract treatment.
Next, to investigate the effect of the Sc extract on xylem development, we stained the xylem structure of the Sc extract-treated Arabidopsis seedlings with Auramine O, a fluorescent dye that mainly stains lignin and suberin31. Under normal growth conditions, the distance from the root tip to the protoxylem tracheary elements was approximately 1000 µm, whereas the distance after AHM or the Sc extract treatment was shortened to ~ 590 µm and ~ 300 µm, respectively (Fig. 5A–G), indicating that both the AHM and the Sc extract have xylem development activity. Intriguingly, additional lignin deposition was observed in the endodermal cells in the root-transition zone after the Sc extract treatment (Fig. 5E,F).
S. chinensis extract induced extended xylem tissue. Florescence image (A) and differential interference contrast (DIC) image (B) of Auramine O-stained root of control (DW) Arabidopsis seedling; florescence image (C) and DIC image (D) of Auramine O-stained root of artificial phytohormone mixture (AHM)-treated Arabidopsis seedling; florescence image (E) and DIC image (F) of Auramine O-stained root of S. chinensis extract (Sc extract)-treated Arabidopsis seedling. Scale bars = 100 µm. (G) Box-and-whisker plots showing the fluorescence intensity in (A) (n = 4 seedlings for each genotype, with three biological replicates). The boxes and solid lines in the boxes show the upper (75th) and lower (25th) quartiles and median values, respectively. Different letters represent significant differences in each point (p < 0.05, Wilcoxon and Steel–Dwass tests). (H) Effect of S. chinensis extract on TE differentiation in cultured hypocotyls cultured with S. chinensis extract. Scale bars = 50 µm. (I) TE differentiation rate. Data are presented as mean ± s.d. n > 20 hypocotyls; **p < 0.01 (ANOVA with Scheffe test).
To further confirm the effects of the Sc extract on xylem vessel formation, segments of the hypocotyl were cultured in xylem vessel-inducing medium32 containing a 0–10% concentrations of the Sc extract stock solution (tenfold dilution of the stock solution). The frequency of xylem vessel formation was elevated at a 1% Sc extract concentration, and was relatively inhibited at concentrations above 2%. The application of up to 5% Sc extract inhibited tracheary element induction, suggesting that the Sc extract can promote or inhibit xylem vessel formation in a dose-dependent manner (Fig. 5H and I).
We also confirmed that basic fuchsin-stained lignified tissues, a component of the secondary cell wall, increased more than fourfold after the Sc extract treatment compared with after the AHM treatment (Fig. 6A and B). Notably, both protoxylem and metaxylem developed into the root elongation zone following the Sc extract treatment, whereas only protoxylem developed after AHM treatment, suggesting that the Sc extract has an ability to induce tracheary elements (Fig. 6B).
S. chinensis extract induced ectopic deposition of the secondary cell wall components in the epidermal cells of the root division zone. (A) Fluorescent and DIC images of 4-day-old Arabidopsis seedlings stained with Alexa Fluor 488-conjugated wheat germ agglutinin (WGA) for secondary cell wall labeling, without or with of the artificial hormone mix (AHM) or S. chinensis extract treatment. Scale bars = 100 µm. (B) Box-and-whisker plots showing the fluorescence intensity in (A) (n = 10 seedlings for each genotype, with three biological replicates). The boxes and solid lines in the boxes show the upper (75th) and lower (25th) quartiles and median values, respectively. Different letters in (B) represent significant differences in each point (P < 0.05, Wilcoxon and Steel–Dwass test). (C) Basic fuchsin staining of the roots of 4-day-old Arabidopsis seedlings without or with AHM solution or S. chinensis extract treatment. Scale bars = 100 µm. (D) Box-and-whisker plot showing the fluorescence intensity in (C) (n = 10 seedlings for each genotype, with three biological replicates). The boxes and solid lines in the boxes show the upper (75th) and lower (25th) quartiles and median values, respectively. Different letters in (D) represent significant differences in each point (p < 0.05, Wilcoxon and Steel–Dwass tests).
Finally, we determined whether the Sc extract could induce secondary cell wall in root-tip region. The secondary cell wall was stained with Alexa Fluor 488-conjugated wheat germ agglutinin (WGA), which binds to the 4-O-methylglucuronic acid of xylan and is an indicator of the secondary cell wall32. After the Sc extract treatment, secondary cell wall emerged in the epidermal cells of the root transition and elongation zone; they were not observed in the control, AHM, A. pisum or M. crassicauda extract treatments (Fig. 6C and D, Fig. S3). Furthermore, we investigated whether the distribution of phytohormones, auxin, and cytokines is altered after the aphid extract treatments using fluorescent reporter lines of auxin (DR5rev::GFP)33 and cytokines (TCS::GFP)34, respectively. Auxin, but not cytokines, was spread following the Sc extract treatment. No changes in the distribution of auxin and cytokines were observed after A. pisum or M. crassicauda extract treatments (Figs. S4 and S5).
Collectively, only the Sc extract treatment induced gall-like structures in Arabidopsis seedlings, comprising secondary cell wall formation in the epidermal cells, the enhancement of xylem cell formation, and the dedifferentiation of epidermal and cortical cells in the root region. In contrast, AHM treatment only enhanced protoxylem elongation, suggesting that effectors other than plant hormones act to induce stem cell niche maintenance, secondary cell wall formation, and xylem cell formation.
RNA-seq analyses of aphid extract and hormone mixture treatments
We performed RNA-seq analysis of Arabidopsis seedlings treated with the Sc extract and AHM to determine whether the changes in the expression of Arabidopsis seedlings are induced by only phytohormones or phytohormones plus other effector molecules in the Sc extract. The Sc extract and AHM treatments upregulated 4129 and 3273, differentially expressed genes (DEGs), respectively. A Venn diagram analysis indicated that of these DEGs, 619, 1,024, and 469 were upregulated in the Sc extract treatment, AHM treatment, and commonly upregulated in these treatments, respectively. In contrast, 770, 1,175, and 356 DEGs were downregulated in the Sc extract treatment, AHM treatment, and commonly downregulated in both treatments, respectively. We performed a gene ontology (GO) enrichment analysis on the commonly upregulated DEG sets in Sc and AHM treatments and found that the upregulated DEGs were categorized into Hormone (GO-term categories: “response to abscisic acid” and “response to jasmonic acid”), Abiotic stress response (GO-term categories: “response to light stimulus”, “response to alcohol”, “response to lipid”, “response to mannose”, “response to water”, “response to oxidative stress”, “response to salt stress”, “response to sucrose”, “response to hypoxia” and “response to osmotic stress”), Development (GO-term categories: “leaf senescence”, “aging”), Metabolite (GO-term categories: “suberin biosynthetic process”, “terpenoid catabolic process” “isoprenoid catabolic process”, “apocarotenoid metabolic process”, “phenylpropanoid biosynthetic process” and “olefinic compound metabolic process”) (Fig. 7, Supplementary Tables S1–S3). Among the 469 commonly upregulated genes, 57 transcription factors were differentially expressed. These transcription factors were categorized as auxin signal transduction, ABA signal transduction, and circadian rhythm (Table S4). As the Sc extract contained IAA and ABA, the upregulation of these transcription factors could be accomplished through the effects of aphid-derived phytohormones. The Ab-GALFA results revealed that the Sc extract treatment induced the dedifferentiation of the epidermal and cortical cells in the transition zone, secondary cell wall formation in the epidermal cells in the differentiation zone, and the enhancement of xylem cell formation in the root. In accordance with these morphological changes, transcriptional regulators of stem cell formation (CLE44, WOX5, and Class-1 KNOXs), xylem development (VND7), and secondary cell wall construction (MYB105, MYB42, MYB45, MYB52, MYB56, and MYB63) were significantly upregulated in the Arabidopsis seedlings treated with the Sc extract (Table 2).
Venn diagram analysis of the number of increased differentially expressed genes (DEGs) in the Arabidopsis seedlings following Schlechtendalia chinensis extract treatment and phytohormone mixture treatment. The number in each circle represents the number of upregulated DEGs in each treatment. The number in the overlapping region indicates the shared DEGs between the two treatments. Descriptions in each region indicate the typically enriched gene ontology categories of the corresponding groups. denotes the corresponding GO-term categories with the RNAseq analysis of the early developmental galls of S. chinensis18.
Thus, although many genes were commonly upregulated in both the Sc extract and AHM treatments, the expression of significant number of genes were altered only in the Sc extract treatment (Fig. 7).
In the case of GO enrichment analysis of downregulated genes of Arabidopsis seedlings treated with Sc extract or AHM. The genes related to the photosynthetic process were downregulated both in the Sc extract and AHM treatments, indicating that the photosynthetic activity was changed by Sc and AHM treatments in chloroplasts of cotyledons, because both of positive and negative regulators are included in GO enrichment analysis. Intriguingly, two categories, “defense response” and “electron transport chain generation of precursor” were related to the genes increased and downregulated only in Sc treatment, as well as in the natural gall of R. javanica. These results suggest that not only phytohormones but also unknown effector molecules in the Sc extract regulated the gene expression of the Arabidopsis seedlings in this treatment.
Discussion
The wide variation in the unique shapes of insect galls has attracted attention. However, the molecular mechanisms underlying gall development remain poorly understood owing to the lack of a gall-inducing insect–host plant model. Gall-inducing insects produce effectors that can control the developmental programs of their host plants. In this study, we found that abnormal structures in the root tip region of A. thaliana induced by an extract of the gall-inducing aphid, S. chinensis have the typical characteristics of the insect gall structures9,10,11.
Typical characteristics of the insect galls is induced by the treatment of S. chinensis extract
We found that the shape of the root region of Arabidopsis seedlings treated with the Sc extract significantly changed. The morphological changes in the root region of the seedlings can be characterized as follows: the essential elements of secondary cell wall (lignin, xylan, and suberin) were deposited in the epidermal cells of the root transition and elongation zone, indicating that secondary cell wall structures were constructed in the secondary cell wall-free cells of the root-transition zone . This characteristic appears to reflect the typical structure of the outer layer of gall tissue.
From the meristematic zone to the elongation zone, the width of the root epidermal cells thickened, while the parallel alignment of the cortical microtubule array was severely disrupted by Sc extract treatment. Furthermore, the bursiform and complex structures of vacuoles in the root division and transition zones changed to more fragmented, smaller vacuolar structures after Sc extract treatment. In addition, a stem cell niche marker (PLT1-GFP or PLT1-mNeonGreen and WOX5mNeonGreen fluorescence) was detected in the epidermal cells of the root-transition zone, in addition to the meristematic cells. From these results, we concluded that the cells of the root-transition zone became dedifferentiated and formed callus-like structures after Sc extract treatment. Furthermore, Sc extract treatment induced xylem vessel differentiation in the root-transition zone. The ability of TE differentiation was also confirmed by an in vitro xylem vessel formation induction system.
Collectively, we concluded that the Sc extract treatment could induce gall-like structures in the root tip region. The gall-like structures are composed of the secondary cell wall in the epidermal cells of the root elongation and differentiation zones, corresponding to the outer shells of galls; the enhancement of xylem vessel formation, corresponding to the newly formed vascular tissues inside galls; the dedifferentiation of epidermal and cortical cells in the root meristematic and elongation regions of the Arabidopsis seedlings, corresponding to the callus-like cells in galls (Fig. 8).
Comparison of the tissue structures and expressed genes of the gall-like structures in Arabidopsis in the root-tip region with Rhus javanica galls. The arrows in the schematic illustration indicate the corresponding tissue structures between R. javanica galls and Arabidopsis gall-like structures. The numbers in the figure represent the log-fold change values of the DEGs compared with each control tissue.
The gall-like structures induced only in the root-tip region are possibly because the cells in the root elongation zone still possess pluripotency. In fact, in root-knot nematode gall formation, the second-stage juvenile (J2) of the root-knot nematodes enters the inner root at the tip, and they migrates intercellularly to the vascular cylinder to reprogram root tissues of the root elongation zone into giant cells35. Moreover, the treatment with J2 crude extracts from outside of the seedlings induces abnormal structures in the root elongation zone36.
Phytohormones in the aphid extract could partially induce gall-like structures in Arabidopsis
Phytohormones produced by gall-inducing insects play key roles in gall formation27. Active phytohormones such as IAA and CKs have been identified in several gall-inducing insects17,27,28. We previously identified amounts of IAA and CK in S. chinensis. In this study, in addition to IAA and CKs6, we observed abscisic acid, SA, and JAs inside the bodies of S. chinensis. Given that these phytohormones in insects are involved in the gall-like structure formation processes, including abnormal cell division, cellular enlargement, and dedifferentiation in Arabidopsis seedlings, the application of an AHM mimicking the phytohormone constituents of the Sc extract might contribute to the induction of the gall-like structures observed in the Arabidopsis seedlings. However, our results showed that the morphological changes (extra xylem vessel formation, the dedifferentiation of epidermal and cortical cells, and the formation of secondary cell wall in the epidermal cells), occurred only by the Sc-extract treatment in the root region of the Arabidopsis seedlings, suggesting the existence of effector molecules other than phytohormones in the Sc extract.
Common characteristics of gene expression profiles of gall formation in S. chinensis and gall-like tissue formation in Arabidopsis
We found several commonly enriched the gene ontology (GO)-term categories of the genes expressed in the developmental gall of Rhus javanica and the gall-like tissue in Arabidopsis root-tip region. The corresponded GO-term categories are “response to hypoxia”, “defense response”, “phenylpropanoid metabolic process”, “response to oxidative stress”, response to osmotic stress”, “electron transport chain”, and “photosynthesis”. These categories are closely related to the typical characteristics of gall tissue of R. javanica6, e.g., the genes categorized to “phenylpropanoid metabolic process” is related to the synthesis of components of secondary cell wall of outer shell of gall tissue, “response to jasmonic acid” and “defense response” are related to the defense reaction of gall-tissue to the biotic stresses, and “response to hypoxia”, “response to oxidative stress”, “response to osmotic stress” are related to the biotic stress reaction of gall-tissue.
Our RNA-seq analysis of Arabidopsis seedlings after treating S. chinensis extract revealed that characteristic genes involved in the formation of the typical gall-structure are significantly increased. For instance, the stem cell maintenance genes, (CLAVATA1, RGFR2, WOX4, and WOX5), a suppressor of plant stem cell differentiation (CLE44), an essential factor for QC specification and stem cell activity (PLT2), root meristem growth factors required for root stem cell niche maintenance (RGF1-RGF4), the vascular development-related genes (ACL5 and VND7), the genes involved in the secondary cell wall biosynthesis (KNAT7, MYB105, MYB42, MYB63, SND2, and SND3) were upregulated by the treatment using of S. chinensis extract (Table 2).
Collectively, we concluded that the expression profile of the gall-like structure induced by the Sc-extract treatment is similar to that of the developmental gall of R. javanica produced by S. chinensis.
Conclusion
In this study, we demonstrated that gall-like structures can be induced in the root regions of Arabidopsis seedlings by the application of an extract of the gall-inducing aphid, S. chinensis. This phenomenon can be used as a model system to analyze the molecular mechanisms of gall formation processes. We referred to this model system as the Arabidopsis-based gall-forming assay (Ab-GALFA). The Ab-GALFA can be utilized as a bioassay for isolating the unknown insect effectors of gall formation in various host plants.
Materials and methods
Plant materials
The A. thaliana ecotype Columbia (Col-0) was used in this study. The transgenic marker lines used for the Ab-GALFA were as follows: PLT1p::PLT1-GFP37, SYP22p::GFP-SYP2222, DR5rev::GFP33, TCS::GFP34, and the microtubule marker line CaMV35Sp::GFP-fused microtubule binding domain (GFP-MBD)21. This study complies with relevant institutional, national, and international guidelines and legislation for using plant material.
Plasmid construction and plant transformation
For the construction of PLT1p::PLT1-GUS-mNeonGreen and WOX5p::WOX5-GUS-mNeonGreen constructs, pGWB533 was digested with SacI, and then mNeonGreen PCR fragment, which was amplified from mNeonGreen vector using the following primers: (533-mNeon-N-f; 5’-cagcagggaggcaaacaaATGGTGAGCAAGGGCGAGGAGG-3′, and 533-mNeon-C-r; 5′-AACGATCGGGGAAATTCGTTACTTGTACAGCTCGTCCATGCC-3′), was ligated into SacI-digested pGWB53338 using NEBuilder HiFi DNA assembly kit (New England Biolabs, Ipswich, MA, U.S.A.) according to manufacturer’s instruction to generate pGWB533-GUS-mNeonGreen. The genomic fragments containing 5′-upstream region (1966 bp) plus the coding region of PLETHORA1 (PLT1) or 5′-upstream region (1908 bp) plus the coding region of WUSCHEL RELATED HOMEOBOX 5 (WOX5) was then inserted into pGWB533-GUS-mNeonGreen via LR reaction (ThermoFisher Scientific, Waltham, MA, U.S.A.) to generate pGWB533-PLT1-GUS-mNeonGreen and pGWB533-WOX5-GUS-mNeonGreen, respectively. The transgenic Arabidopsis lines expressing PLT1-GUS-mNeonGreen or WOX5-GUS-mNeonGreen under control of each own-promoter were generated using Agrobacterium tumefaciens strain GV3101 by the floral dip method39.
Growth conditions
The Arabidopsis seeds were sterilized with 70% ethanol and grown on half-strength Murashige and Skoog salt (1/2 MS) agar medium, supplemented with 1% sucrose, at 22 °C under a 16/8 h light/dark cycle. The 4-day-old seedlings were used for the Ab-GALFA.
Arabidopsis-based gall formation assay (Ab-GALFA)
Approximately 10 S. chinensis bodies isolated from the rapid growth phase of R. javanica gall, and 10 bodies of non-galling aphids Acythosiphon pisum and Megoura crassicauda were frozen in liquid nitrogen, ground to a fine powder, and suspended in 50 µL of sterile distilled water (DW), then the suspensions were centrifuged at 20,000 × g for 5 min at 4 °C to prepare the S. chinensis (Sc) extract stock solution. Arabidopsis seedlings grown vertically on 1/2 MS medium with 1.2% agarose for 4 days were gently removed from the agarose surface using micropipette tips, placed in a Petri dish, and the entire bodies of seedlings were incubated with different concentrations of Sc, Ap or Mc extracts or sterilized water (a negative control) under normal growth conditions for ≥ 15 h. Following incubation, the seedlings were used for subsequent analyses. The working solution of aphid extracts was prepared by diluting the stock solution.
Quantitative analysis of various phytohormones in S. chinensis
The endogenous levels of IAA, cytokinins, abscisic acid, and JAs in S. chinensis bodies were quantitatively analyzed as described by Yamane et al.40, with minor modifications. Briefly, aphid bodies (approximately 100–200 mg per sample) were collected, frozen in liquid nitrogen, and weighed. Then, the samples were ground and subjected to extraction in 80% acetonitrile and 1% acetic acid containing stable isotope-labeled compounds as internal standardsv[13C6-IAA, D5-tZ, D6-iP, D6-ABA, D4-SA (OlChemim, Olomouc, Czech Republic), D5-JA (CDN Isotopes, Quebec, Canada), and 13C6-JA-Ile (kindly gifted by Dr. Yusuke Jikumaru, Riken, Japan; present affiliation: Agilent Technologies Japan, Ltd.)]. After purification of the HLB and MCX columns (Waters Corporation, Milford, MA, USA), phytohormones were analyzed using a 6410 Triple Quad LC/MS system (Agilent Technologies Inc., Santa Clara, CA, USA) equipped with a ZORBAX Eclipse XDB-C18 column and an XDB-C8 Guard column (Agilent Technologies). Peak areas were determined using MassHunter Workstation software (vB.04.00; Agilent Technologies). Four independent samples were analyzed to calculate the averages and standard deviations.
Preparation of the phytohormone mixture solution
According to the results of the S. chinensis phytohormone content analyses, the concentrations in the Artificial Hormone Mixture (AHM) were as follows: 27.0 ng/mL IAA, 17.4 ng/mL ABA, 2.78 ng/mL SA, 0.43 ng/mL JA, and 0.086 ng/mL cytokinin in DW.
Histological staining of Arabidopsis seedlings
The cell walls of the root cells were stained with propidium iodide, as previously described35. To stain the components of the secondary cell wall, the Arabidopsis seedlings were washed in sterilized water and incubated in 1 μg/mL WGA-Alexa Fluor 488 (ThermoFisher Scientific, Waltham, MA, U.S.A.) in sterilized water at 25 °C for 1 h and washed five times for 30 min for each wash. Lignin and suberin were stained with basic fuchsin and Auramine O, respectively, as described by Ursache et al.31.
Image analysis
Confocal fluorescence and differential interference contrast images were obtained using a Leica TCS SP8 laser-scanning confocal microscope (Leica Microsystems, Wetzlar, Germany). The settings of excitation and emission for the observation of each fluorescent dye were as follows: propidium iodide, 555 nm/557–700 nm; GFP, 488 nm/493–547 nm; WGA-Alexa Fluor 488, 488 nm/493–547 nm; basic fuchsin, 552 nm/557–700 nm; and Auramine O 488 nm/493–547 nm. The captured images were processed using Leica LAS X software (Leica).
Measurements of cell length, width, and microtubule density
After staining the root cells with propidium iodide, the length and width of the cells was determined using ImageJ software. The microtubule density in the GFP-TUB6-expressing line with or without the Sc-extract treatment was measured, as previously described41.
Measurements of intensity and statistical analyses
Subsets of data with defined x-, y-, and z-dimensions were acquired using LASX software (Leica), and all subsets were transformed into 2D images using the maximum intensity projection function of LASX software. In all images, uniform brightness and contrast correction was performed before being exported for image analysis. All quantitative data were produced using the publicly available software Image Studio (LI-COR). The values were normalized relative to the average fluorescence intensity measured in the control group. Final statistical data evaluation and plot preparation were performed using R software.
RNA extraction, library construction, and RNA sequencing
Total RNA was extracted from Arabidopsis seedlings using the RNeasy Plant Mini Kit (Qiagen, Hilden, Germany). All samples were pretreated with DNase I, using the Qiagen RNase-Free DNase Set (Qiagen, Hilden, Germany), to eliminate DNA contamination. RNA quality was checked by determining the RNA integrity number using an RNA 6000 bioanalyzer and RNA Nano Chip (Agilent Technologies). RNA-seq libraries were prepared using the Illumina TruSeq® Stranded RNA LT kit (Illumina, San Diego, CA, USA) according to the manufacturer’s instructions. Three independent RNA samples from each tissue were used for the analysis. Pooled libraries were sequenced using NextSeq 500 (Illumina), and single-end reads, 75 bp in length, were obtained. The obtained reads were mapped to the reference A. thaliana genome (TAIR10) using TopHat242. The htseq-count script in the HTSeq library was used to count the reads43. Count data were subjected to trimmed mean of M-value normalization using EdgeR44,45. DEGs were defined using the EdgeR GLM approach45, and genes with FDRs < 0.05 were classified as DEGs. Scaled expression values were used for clustering, based on the SOM46,47.
In vitro induction assay for xylem vessel formation
The xylem vessel cell differentiation assay have previously been described32. Briefly, A. thaliana (Col-0) seedlings were grown on MS solid medium containing 2.3 g/L MS salt mix (FUJIFILM Wako Chemicals, Osaka, Japan), 10 g/L sucrose, 0.5 mg/L nicotinic acid, 0.1 mg/L thiamine HCl, 0.5 mg/L pyridoxine HCl, 0.1 g/L myo-inositol, 2 mg/L glycine (pH 5.8), and 5 g/L phytagel (Sigma-Aldrich, St Lousis, MO, U.S.A. ) under dark conditions for 5 d after a 2-day-long vernalization period. Hypocotyls (25–30) were cut and transferred into 1-mL aliquots of TE-inducing medium (liquid MS medium) supplemented with 0.1 mg/L 2,4-D, 1 mg/L kinetin, and 10 µM bikinin, together with 0–10% (diluted with DW) Sc extract in 12 multi-well plates. The hypocotyls were cultured on a rotary shaker at 60 rpm for 8 days at 21 °C.
Data availability
The datasets generated for this study can be found in the DDBJ as the BioProject Submission IDs; PRJDB13617 (https://ddbj.nig.ac.jp/resource/bioproject/PRJDB13617I) and PRJDB13618 (https://ddbj.nig.ac.jp/resource/bioproject/PRJDB13618). All data and materials appearing in this study are available from the corresponding author (M.H.S.) upon reasonable request.
References
Stone, G. N. & Scho, K. The adaptive significance of insect gall morphology. Trends Ecol. Evol. 18, 512–522 (2003).
Giron, D., Huguet, E., Stone, G. N. & Body, M. Insect-induced effects on plants and possible effectors used by galling and leaf-mining insects to manipulate their host-plant. J. Insect Physiol. 84, 70–89 (2016).
Rohfritsch, O. & Shorthouse, J. D. Insect galls. In Molecular Biology of Plant Tumors (eds Kahl, G. & Schell, J.) 121–152 (Academic Press, 1982).
Kurosu, U. & Aoki, S. Formation of a ‘Cat’s-paw’ gall by the aphid Ceratovacuna nekoashi (Homoptera). Jpn. J. Entomol. 58, 155–166 (1990).
Schultz, J. C., Edger, P. P., Body, M. J. M. & Appel, H. M. A galling insect activates plant reproductive programs during gall development. Sci. Rep. 9, 1833 (2019).
Hirano, T. et al. Reprogramming of the developmental program of Rhus javanica during initial stage of gall induction by Schlechtendalia chinensis. Front. Plant Sci. 11, 471 (2020).
Takeda, S. et al. Comparative transcriptome analysis of galls from four different host plants suggests the molecular mechanism of gall development. PLoS ONE 14, e0223686 (2019).
Takeda, S., Hirano, T., Ohshima, I. & Sato, M. H. Recent progress regarding the evolution and molecular aspect of insect gall formation. Int. J. Mol. Sci. 22, 9424 (2021).
Mani, M. S. Ecology of Plant Galls (W. Junk, 1964).
Guiguet, A., Ohshima, I., Takeda, S., Laurans, F. & Giron, D. Origin of gall-inducing from leaf- mining in Caloptilia micromoths. Sci. Rep. 9, 6794 (2019).
Guiguet, A. et al. Inside the horn of plenty: Leaf-mining micromoth manipulates its host plant to obtain unending food provisioning. PLoS ONE 13, e0209485 (2018).
Barnewall, E. & De Clerck-Floate, R. A. A preliminary histological investigation of gall induction in an unconventional galling system. Arthropod. Plant. Interact. 6, 449–459 (2012).
Yamaguchi, H. et al. Phytohormones and willow gall induction by a gall-inducing sawfly. New Phytol. 196, 586–595 (2012).
Tooker, J. F., De Moraes, C. M. & De Moraes, C. M. Feeding by a gall-inducing caterpillar species alters levels of indole-3-acetic and abscisic acid in Solidago altissima (Asteraceae) stems. Arthropod. Plant. Interact. 5, 115–124 (2011).
Mapes, C. C. & Davies, P. J. Indole-3-acetic acid and ball gall development on Solidago altissima. New Phytol. 151, 195–202 (2001).
Straka, J. R., Hayward, A. R. & Emery, R. J. N. Gall-inducing Pachypsylla celtidis (Psyllidae) infiltrate hackberry trees with high concentrations of phytohormones. J. Plant Interact. 5, 197–203 (2010).
Tanaka, Y., Okada, K., Asami, T. & Suzuki, Y. Phytohormones in japanese mugwort gall induction by a gall-inducing gall midge. Biosci. Biotechnol. Biochem. 77, 1942–1948 (2013).
Pascual-Alvarado, E., Cuevas-Reyes, P., Quesada, M. & Oyama, K. Interactions between galling insects and leaf-feeding insects: The role of plant phenolic compounds and their possible interference with herbivores. J. Trop. Ecol. 24, 329–336 (2008).
Eng, R. C. & Sampathkumar, A. Getting into shape: The mechanics behind plant morphogenesis. Curr. Opin. Plant Biol. 46, 25–31 (2018).
Cui, Y., Zhao, Q., Hu, S. & Jiang, L. Vacuole biogenesis in plants: How many vacuoles, how many models?. Trends Plant Sci. 25, 538–548 (2020).
Marc, J. et al. A GFP–MAP4 reporter gene for visualizing cortical microtubule rearrangements in living epidermal cells. Plant Cell 10, 1927–1939 (1998).
Uemura, T., Yoshimura, S. H., Takeyasu, K. & Sato, M. H. Vacuolar membrane dynamics revealed by GFP-AtVam3 fusion protein. Genes Cells 7, 743 (2002).
Oda, Y., Higaki, T., Hasezawa, S. & Kutsuna, N. New insights into plant vacuolar structure and dynamics. Int. Rev. Cell Mol. Biol. 277, 103 (2009).
Zheng, H. & Andrew Staehelin, L. Protein storage vacuoles are transformed into lytic vacuoles in root meristematic cells of germinating seedlings by multiple, cell type-specific mechanisms. Plant Physiol. 155, 2023–2035 (2011).
Segami, S., Makino, S., Miyake, A., Asaoka, M. & Maeshima, M. Dynamics of Vacuoles and H+-pyrophosphatase visualized by monomeric green fluorescent protein in Arabidopsis: Artifactual bulbs and native intravacuolar spherical structures. Plant Cell 26, 3416–3434 (2014).
Tooker, J. F. & Helms, A. M. Phytohormone dynamics associated with gall insects, and their potential role in the evolution of the gall-inducing habit. J. Chem. Ecol. 40, 742–753 (2014).
Mapes, C. C. & Davies, P. J. Cytokinins in the ball gall of Solidago altissima and in the gall forming larvae of Eurosta solidaginis. New Phytol. 151, 203–212 (2001).
Bartlett, L. & Connor, E. F. Exogenous phytohormones and the induction of plant galls by insects. Arthropod Plant Interact. 8, 339–348 (2014).
Aida, M. et al. The PLETHORA genes mediate patterning of the Arabidopsis root stem cell niche. Cell 119, 109–120 (2004).
Sarkar, A. K. et al. Conserved factors regulate signalling in Arabidopsis thaliana shoot and root stem cell organizers. Nature 446, 811–814 (2007).
Ursache, R. et al. A protocol for combining fluorescent proteins with histological stains for diverse cell wall components. Plant J. 93, 399–412 (2017).
Sasaki, T. & Oda, Y. Imaging of developing metaxylem vessel elements in cultured hypocotyls. Methods Mol. Biol. 1992, 351–358 (2019).
Friml, J. et al. Efflux-dependent auxin gradients establish the apical-basal axis of Arabidopsis. Nature 426, 147–153 (2003).
Zürcher, E. et al. A robust and sensitive synthetic Ssensor to monitor the transcriptional output of the cytokinin signaling network in planta. Plant Physiol. 161, 1066–1075 (2013).
Sato, K., Kadota, Y. & Shirasu, K. Plant immune responses to parasitic nematodes. Front. Plant Sci. 10, 1165 (2019).
Teixeira, M. A., Wei, L. & Kaloshian, I. Root-knot nematodes induce pattern-triggered immunity in Arabidopsis thaliana roots. New Phytol. 211, 276–287 (2016).
Matsuzaki, Y., Ogawa-Ohnishi, M., Mori, A. & Matsubayashi, Y. Secreted peptide signals required for maintenance of root stem cell niche in Arabidopsis. Science 329, 1065–1067 (2010).
Nakagawa, T. et al. Development of series of gateway binary vectors, pGWBs, for realizing efficient construction of fusion genes for plant transformation. J. Biosci. Bioeng. 104, 34–41 (2007).
Clough, S. J. & Bent, A. F. Floral dip: a simplified method for Agrobacterium-mediated transformation of Arabidopsis thaliana. Plant J. 16, 735–743 (1998).
Yamane, H. et al. Overexpression of Prunus DAM6 inhibits growth, represses bud break competency of dormant buds and delays bud outgrowth in apple plants. PLoS ONE 14, e0214788 (2019).
Hirano, T. et al. PtdIns (3,5)P2 mediates root hair shank hardening in Arabidopsis. Nat. Plants 4, 888–897 (2018).
Kim, D. et al. TopHat2: accurate alignment of transcriptomes in the presence of insertions, deletions and gene fusions. Genome Biol. 14, R36 (2013).
Anders, S., Pyl, P. T. & Huber, W. HTSeq-A Python framework to work with high-throughput sequencing data. Bioinformatics 31, 166–169 (2015).
Robinson, M. D., McCarthy, D. J. & Smyth, G. K. edgeR: A Bioconductor package for differential expression analysis of digital gene expression data. Bioinformatics 26, 139–140 (2009).
McCarthy, D. J., Chen, Y. & Smyth, G. K. Differential expression analysis of multifactor RNA-Seq experiments with respect to biological variation. Nucleic Acids Res. 40, 4288–4297 (2012).
Kohonen, T. Self-organized formation of topologically correct feature maps. Biol. Cybern. 43, 59–69 (1982).
Wehrens, R. & Buydens, L. M. C. Self- and super-organizing maps in R: The kohonen package. J. Stat. Softw. 21, 1–19 (2007).
Acknowledgements
We thank Ms. Kaori Kaminoyama (Kyoto Sangyo University) for technical help for library construction and RNA–sequencing. This work was supported by JSPS KAKENHI a-Grant-in-Aid for Scientific Research (A) (Grant No. 21K06234) to M.H.S., and a-Grant-in-Aid for Scientific Research (C) (Grant No. 19H00933) to S.T., by the strategic prioritized research in KPU to M.H.S. and S.T., by JST, PRESTO Grant Number JPMJPR20D5, Japan to T. Hirano, by JSPS KAKENHI Grant, grant No. 21H02513 to S.K., by the MEXT-Supported Program for the Strategic Research Foundation at Private Universities, grant No. S1511023 to S.K.
Author information
Authors and Affiliations
Contributions
T.Hirano. and M.H.S. conceived and designed the study. T.Hirano. and A.O. performed the image analysis. T.Higaki. performed the measurement of the microtubule density. T.M., S.T. and Y.I. measured the content of various phytohormones in S. chinensis. T.S. and S.K. constructed library and performed RNA-sequencing. T.Hirano. and M.H.S. wrote the manuscript. M.H.S. supervised the project.
Corresponding author
Ethics declarations
Competing interests
The authors declare no competing interests.
Additional information
Publisher's note
Springer Nature remains neutral with regard to jurisdictional claims in published maps and institutional affiliations.
Supplementary Information
Rights and permissions
Open Access This article is licensed under a Creative Commons Attribution 4.0 International License, which permits use, sharing, adaptation, distribution and reproduction in any medium or format, as long as you give appropriate credit to the original author(s) and the source, provide a link to the Creative Commons licence, and indicate if changes were made. The images or other third party material in this article are included in the article's Creative Commons licence, unless indicated otherwise in a credit line to the material. If material is not included in the article's Creative Commons licence and your intended use is not permitted by statutory regulation or exceeds the permitted use, you will need to obtain permission directly from the copyright holder. To view a copy of this licence, visit http://creativecommons.org/licenses/by/4.0/.
About this article
Cite this article
Hirano, T., Okamoto, A., Oda, Y. et al. Ab-GALFA, A bioassay for insect gall formation using the model plant Arabidopsis thaliana. Sci Rep 13, 2554 (2023). https://doi.org/10.1038/s41598-023-29302-8
Received:
Accepted:
Published:
DOI: https://doi.org/10.1038/s41598-023-29302-8
Comments
By submitting a comment you agree to abide by our Terms and Community Guidelines. If you find something abusive or that does not comply with our terms or guidelines please flag it as inappropriate.