INTRODUCTION
Heart failure (HF) can be defined physiologically as an inability of the heart to provide sufficient forward output to meet the perfusion and oxygenation requirements of the tissues while maintaining normal filling pressures. Chronic heart failure can be divided into two broad categories: systolic heart failure (SHF) and diastolic heart failure (DHF). Classifying patients in these two broad categories should be based on characteristic changes in cardiovascular structure and function (see Chapter 28 ).
SHF is characterized by progressive chamber dilation, eccentric remodeling, and dominant abnormalities in systolic properties. Clinical manifestations of left ventricular (LV) systolic dysfunction include decreased cardiac output, increased heart rate, and peripheral vasoconstriction. However, patients with SHF frequently have additional symptoms of shortness of breath at rest or with exertion. These symptoms of pulmonary congestion are due, at least in part, to LV diastolic dysfunction. Therefore, patients with SHF (particularly when they have symptomatic decompensation) do not have an “isolated” abnormality in systolic properties; rather, from the pathophysiologic point of view, they have predominant abnormalities in systolic properties and eccentric remodeling, with associated or secondary abnormalities in diastolic function.
By contrast, patients with DHF are characterized by normal LV volume, concentric remodeling, and normal LV chamber systolic properties, but dominant abnormalities in diastolic properties. These patients have abnormalities in diastolic relaxation, filling, and/or distensibility. Clinical manifestations of LV diastolic dysfunction include shortness of breath at rest or with exertion and peripheral edema. However, abnormalities in regional systolic shortening have also been identified in some patients with DHF. Therefore, patients with DHF do not have “isolated” abnormalities in diastolic properties; rather, from the pathophysiologic point of view, they have predominant abnormalities in diastolic properties and concentric remodeling.
“Diastolic dysfunction” and “diastolic heart failure” are not synonymous terms. Diastolic dysfunction indicates a functional abnormality of diastolic relaxation, filling, or distensibility of the left ventricle—regardless of whether the ejection fraction is normal or abnormal and regardless of whether the patient is asymptomatic or has symptoms and signs of HF. Thus, diastolic dysfunction comprises abnormal mechanical (diastolic) properties of the ventricle and is present in virtually all patients with heart failure. Diastolic heart failure denotes signs and symptoms of clinical heart failure with normal ejection fraction and LV diastolic dysfunction. Similar distinctions apply to the terms “systolic dysfunction” and “systolic heart failure” ( Boxes 2-1 and 2-2 ).
Diastolic Dysfunction: Abnormal diastolic properties of LV (abnormal relaxation, filling dynamics, distensibility)
- •
EF may be normal or low.
- •
Patient may be symptomatic or asymptomatic.
Diastolic Heart Failure: Clinical heart failure, normal ejection fraction, abnormal diastolic function
Systolic Dysfunction: Abnormal systolic properties of LV (abnormal performance, function, contractility)
- •
EF is low (and diastolic dysfunction may coexist).
- •
Patient may be symptomatic or asymptomatic.
Systolic Heart Failure: Clinical heart failure, low ejection fraction, abnormal systolic function (From Circulation 2006;113:296–304.)
Required Criteria
- 1.
Clinical evidence of heart failure
- •
Framingham or Boston criteria
- •
Plasma brain natriuretic peptide and/or chest x-ray
- •
Cardiopulmonary exercise testing
- •
- 2.
Normal ejection fraction (= 50%)
Confirmatory Evidence
- 1.
LVH or concentric remodeling
- 2.
Left atrial enlargement (in absence of atrial fibrillation)
- 3.
Echo Doppler or catheter evidence of diastolic dysfunction
Exclusion
Nonmyocardial disease
PATHOPHYSIOLOGY
The pathophysiology of diastolic heart failure will be reviewed here, beginning with a discussion of the factors involved in normal diastolic relaxation and filling. Understanding normal diastolic function permits an easier understanding of some of the clinical features of DHF.
Normal Diastolic Function
Cardiac function is critically dependent upon diastolic physiologic mechanisms to provide adequate LV filling (cardiac input) in parallel with LV ejection (cardiac output) both at rest and during exercise. Adequate pulmonary function is also dependent upon LV diastolic function. During diastole, the left ventricle, left atrium, and pulmonary veins form a “common chamber” that is continuous with the pulmonary capillary bed. LV diastolic pressure is determined by the volume of blood in the left ventricle during diastole and the diastolic distensibility or compliance of the entire cardiovascular system, principally the left ventricle (but it may also include the left atrium, pulmonary vessels, right ventricle, and systemic arteries). Thus, an increase in LV diastolic pressure (whether this occurs at rest or during exercise) will increase pulmonary capillary pressure, which, if high enough, causes dyspnea, exercise limitation, pulmonary congestion, and edema ( Fig. 2-1 ).

Relaxation of the contracted myocardium begins at the onset of diastole. This is a dynamic process that takes place during isovolumic relaxation (the period between aortic valve closure and mitral valve opening during which LV pressure declines with no change in volume) and then continues during auxotonic relaxation (the period between mitral valve opening and mitral valve closure during which the left ventricle fills at variable pressure) ( Fig. 2-2 ). The rapid pressure decay and the concomitant “untwisting” and elastic recoil of the left ventricle produce a suction effect that augments the left atrial-ventricular pressure gradient and pulls blood into the ventricle, thereby promoting diastolic filling. During exercise in normal patients, relaxation rate is increased and early diastolic pressures decrease, augmenting elastic recoil and diastolic suction and resulting in more rapid filling during a shortened diastolic filling period at increasing heart rates ( Fig. 2-3 ).


During the later phases of diastole, the normal left ventricle is composed of completely relaxed cardiomyocytes and is very compliant and easily distensible, offering minimal resistance to LV filling over a normal volume range. Atrial contraction near the end of diastole contributes 20%–30% to total LV filling volume and increases diastolic pressures by less than 5 mmHg. As a result, LV filling can normally be accomplished by very low filling pressures in the left atrium and pulmonary veins, preserving a low pulmonary capillary pressure (<12 mmHg) and a high degree of lung distensibility. Loss of normal LV diastolic relaxation and distensibility, from structural and functional causes, impairs LV pressure decline and filling, resulting in increases in LV diastolic, left atrial (LA), and pulmonary venous pressures, which directly increase pulmonary capillary pressure.
Measurements of Diastolic Function
Complete characterization of LV diastolic properties requires simultaneous measurement (often using a high-fidelity micromanometer) of LV diastolic pressure and LV volume using invasive and/or noninvasive methods. Diastolic function can be assessed using the following measurements:
- □
Rate of isovolumic relaxation: peak (-)dP/dt, the time constant of the isovolumic LV pressure decay (Tau) and the isovolumic relaxation time (IVRT). When relaxation rate is decreased, (-)dP/dt and Tau are increased ( Fig. 2-4 ).
Figure 2-4
Left ventricular (LV) isovolumic relaxation. LV pressure versus time and the rate of change of LV pressure (dP/dt) in a normal subject ( solid line ) versus a patient with diastolic heart failure ( dashed line ). Isovolumic relaxation can be quantified by calculating the peak instantaneous rate of LV pressure decline (peak –dP/dt) and the time constant of LV isovolumic pressure decline (Tau). When the natural log of LV diastolic pressure is plotted versus time, Tau equals the slope of this linear relationship ( right panel ). Stated in more conceptual terms, Tau is the time required for LV pressure to fall by approximately two thirds of its initial value. When isovolumic relaxation is abnormal, LV pressure decline is slower, –dP/dt has a more negative value; the time constant Tau is prolonged, and its numerical value is increased (depicted by the dashed lines in both figure panels).
- □
Rate and extent of LV filling: filling rate, the time-to-peak filling rate (TPFR), transmitral flow velocity, tissue velocity, and strain and strain rate. When there is prolonged relaxation, the early filling rate and extent are decreased, TPFR is prolonged, and the filling rate and extent that results from atrial contraction are increased ( Fig. 2-5 ).
Figure 2-5
Left ventricular (LV) filling dynamics. LV volume versus time and the rate of change of LV volume (dV/dt) in a normal subject ( solid line ) versus a patient with diastolic heart failure ( dashed line ). With the onset of systole, the LV volume decreases and reaches its minimum at the end systole. Diastolic filling has three distinct phases: rapid filling of the left ventricle, during which dV/dt reaches it maximum and the peak filling rate occurs; a slow filling phase (diastasis), during which there is little change in LV volume; and an atrial systole phase, during which active atrial contraction fills the left ventricle and allows it to attain its end diastolic volume. Important diastolic parameters include the peak filling rate, the time to peak filling rate, the rapid filling fraction (percent of total stroke volume reached during rapid filling), and the percent contribution of atrial systole to LV filling.
- □
Passive elastic stiffness properties: diastolic pressure-volume (P-V) relationship. When stiffness is increased (distensibility is decreased) the diastolic pressure versus volume relationship is shifted upward ( Fig. 2-6 ).
Figure 2-6
Diastolic pressure versus volume relationships in patients with heart failure. Left ventricular (LV) diastolic pressure-volume data from normal controls ( solid line ), patients with diastolic heart failure (DHF) ( dotted line ), and patients with systolic heart failure (SHF) ( dashed line ). In patients with DHF, the diastolic pressure-volume curve is shifted up and left, indicating an increase in passive stiffness and a decrease in distensibility of the left ventricle. In contrast, in patients with SHF, the diastolic pressure-volume curve is shifted down and right, indicating a decrease in passive stiffness and an increase in distensibility of the left ventricle. These data clearly indicate that all patients with heart failure, whether diastolic or systolic, have a significant increase in LV diastolic pressure; however, the mechanisms responsible for these increased pressures are different between these two different patient groups.
(From Aurigemma GP et al: Contractile behavior in the left ventricle in diastolic heart failure: With emphasis on regional systolic function. Circulation 2006;113:296.)
Left Ventricular Pressure Decline
Isovolumic relaxation can be quantified by measurement of LV pressure with a high-fidelity micromanometer catheter and calculation of the peak instantaneous rate of LV pressure decline (peak – dP/dt) and the time constant of LV isovolumic pressure decline (Tau). When the natural log of LV diastolic pressure is plotted versus time, Tau equals the slope of this linear relationship. Stated in more conceptual terms, Tau is the time required for LV pressure to fall by approximately two thirds of its initial value. When isovolumic pressure decline is slowed, Tau is prolonged and its numerical value increases. Noninvasive estimates of the total IVRT can be made using echocardiographic techniques. However, no index of relaxation (isovolumic or auxotonic) can be considered an index of “intrinsic” relaxation rate unless loading conditions (and other modulators) are held constant or are at least specified. Therefore, changes in afterload (systolic pressure) and diastolic load (LA diastolic pressures) may change measurements of isovolumic and auxotonic relaxation without changing intrinsic relaxation properties.
Left Ventricular Filling
A detailed description of the Doppler echocardiographic assessment of diastolic dysfunction is provided in other ( chapter 10 , chapter 11 , chapter 12 and 15 ). The normal left ventricle has a characteristic pattern of filling and inflow velocities. LV inflow velocity and the rate of LV filling are greatest early (E) in diastole, immediately after mitral valve opening, and are responsible for the normally tall E wave of the transmitral inflow Doppler echocardiogram ( Fig. 2-7 ). Since most atrial-to-ventricular transfer of blood occurs in early and mid-diastole, the amount of blood transported by atrial contraction is relatively small, the velocity imparted by the atrial contraction (the A wave of the transmitral inflow Doppler echocardiogram) is relatively low, and the normal E/A wave ratio is greater than 1 and approaches a value of 2 in younger individuals.

Doppler echocardiographic assessment of LV filling has limitations, since diastolic filling parameters are influenced by multiple factors, the most important of which are loading conditions. The typical, but nonspecific, mitral filling pattern associated with diastolic dysfunction (termed abnormal relaxation) is a pattern of increased IVRT and decreased E/A ratio. However, this pattern can be altered or pseudonormalized by changes in LA pressure. When diastolic dysfunction occurs, relaxation is slowed and incomplete, early LV diastolic pressures rise, early diastolic suction falls, and LV filling becomes increasingly dependent on an increase in LA pressure to push blood into the left ventricle during diastole. As LA pressures rise, the value of the E wave increases and E/A increases to a “normal” (or pseudonormal) value. When LA pressures are severely increased, a “restrictive” pattern may develop in which the IVRT may be decreased and the E/A ratio further increased. Recently developed echocardiographic and Doppler echocardiographic techniques can be used to distinguish these three patterns of abnormal LV filling.
When transmitral Doppler flow patterns are examined in concert with more recently developed Doppler echocardiographic techniques, patterns of normal versus impaired relaxation versus pseudonormal versus restriction can be determined because these newer techniques provide information about LV filling pressure and the LA/LV diastolic pressure gradient. These techniques include measuring pulmonary venous flow velocity, tissue Doppler myocardial velocity, strain and strain rate, and color M-mode flow acceleration patterns. In particular, measures of myocardial velocities made by tissue Doppler imaging (TDI) appear less sensitive to alteration in LV loading conditions ( Fig. 2-8 ). E′ measures the rate of early diastolic myocardial lengthening and, when combined with transmitral Doppler E-wave data, can be used to estimate pulmonary capillary wedge pressure (PCWP) = 2 + 1.3 (E/E′).

Left Ventricular Stiffness/Distensibility
LV ventricular diastolic stiffness and distensibility are quantified by the position and shape of the LV diastolic P-V relationship, which plots LV diastolic pressure as a function of LV diastolic volume throughout diastole (see Fig. 2-6 ). A relatively stiff, nondistensible ventricle will require higher pressures to achieve filling of a given volume. Thus, an increase in LV diastolic chamber stiffness or a decrease in distensibility shifts the LV diastolic P-V curve upward and often increases its slope.
Defining the entire LV filling curve throughout diastole requires the simultaneous measurement of LV diastolic pressure and volume either throughout a single cardiac cycle (to define the diastolic P-V relationship) or by way of the end diastolic P-V coordinate over variably loaded cardiac cycles (to define the end diastolic P-V relationship). The volume measurements can be made by angiography, echocardiography, or radionuclide imaging techniques simultaneous with invasive measurements of LV diastolic pressure. Alternatively, noninvasive Doppler echocardiographic techniques can be used to estimate PCWP (see earlier description). Together with echocardiographically measured end diastolic volume, an index of instantaneous diastolic stiffness (ratio of PCWP-to-end diastolic volume) can be derived.
Left Ventricular Diastolic Function During Exercise
A detailed description of the mechanisms and clinical relevance of diastolic dysfunction during exercise is provided in Chapter 17 . The cardiac output can increase several fold during exercise, an appropriate response to the enhanced needs of exercising muscle. Multiple factors contribute to this response, including an increase in heart rate, a modest rise in stroke volume, a reduction in peripheral vascular resistance, and an elevation in contractile force, which increases LV, arterial systolic pressure, and the force of ejection.
The increase in LV output must be matched by a rise in LV input. The left ventricle cannot accomplish this task by the same mechanisms that increase output during exercise. For example, tachycardia shortens the duration of diastole, the time during which LV filling must occur. As a result, the diastolic filling rate during exercise may be increased out of proportion to cardiac output.
A rise in flow rate across the mitral valve requires an increase in the transmitral diastolic pressure gradient. If this were achieved by increasing the pressure in the left atrium, this would have the deleterious effects of increasing pulmonary capillary pressure and causing pulmonary congestion, dyspnea, and respiratory compromise. Rather, the normal left ventricle permits a remarkable increase in diastolic filling rate during exercise by rapidly and markedly decreasing LV pressure during early diastole, thereby creating a relative LV “suction” effect, which enhances the transmitral pressure gradient without increasing LA pressure (see Fig. 2-3 ).
Several mechanisms contribute to the left ventricular diastolic suction effect during exercise:
- □
The increased force of contraction during systole enhances early diastolic myocardial elastic recoil due to greater systolic shortening forces and the extent of systolic fiber shortening, which is manifested as a smaller end systolic volume (ESV). Thus, an increase in systolic shortening results in an increase in restoring forces during diastole, which allow enhanced diastolic filling.
- □
Acceleration of myocyte relaxation occurs during exercise, due to an increased rate of calcium uptake by the sarcoplasmic reticulum (SR). Increased cyclic adenosine monophosphate (cAMP), generated by the β-adrenergic response to exercise, phosphorylates the regulatory SR membrane protein, phospholamban, to increase the rate of calcium uptake by the SR during diastole.
Some of the mechanisms that allow an increase in cardiac output and in cardiac input during exercise act in concert on systolic and diastolic functions:
- □
The Treppe effect creates a relationship between the heart rate (or frequency of contraction)/LV pressure (or systolic force development) and ejection fraction (or shortening) such that in a normal heart, an increase in heart rate is associated with an increase in stroke volume over a physiologic range of heart rate. This has been called the systolic force-frequency relationship. In addition, the same mechanism governs the relationship between heart rate and diastolic relaxation rate where increased heart rate in a normal heart is associated with an increased relaxation rate, which in part allows LV diastolic pressures and PCWP to remain normal during exercise.
- □
A second example of the determinants of systolic and diastolic function acting in concert during exercise is the relationship between increased stroke volume and diastolic distensibility. During normal exercise, end systolic volume decreases and end diastolic volume increases. The increase in end diastolic volume allows the left ventricle to use the Frank-Starling mechanism to augment stroke volume. However, it is the normal stiffness and distensibility of the left ventricle that allows an increase in end diastolic volume with a negligible change in late diastolic pressure and no significant change in PCWP.
In summary, the normal heart during exercise has an elegant balance of physiologic mechanisms to ensure that cardiac input keeps pace with cardiac output, with preservation of a low pulmonary capillary pressure. These mechanisms result in an increase in measured LV distensibility, as manifested by a downward shift of the LV diastolic P-V curve, especially during early diastole ( Fig. 2-9 ).
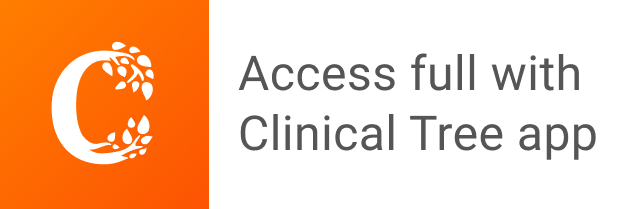