- Department of Oncology, Shengjing Hospital of China Medical University, Shenyang, China
mRNA products are therapies that are regulated from the post-transcriptional, pre-translational stage of a gene and act upstream of protein synthesis. Compared with traditional small molecule drugs and antibody drugs, mRNA drugs had the advantages of simple design, short development cycle, strong target specificity, wide therapeutic field, and long-lasting effect. mRNA drugs were now widely used in the treatment of genetic diseases, tumors, and viral infections, and are expected to become the third major class of drugs after small molecule drugs and antibody drugs. The delivery system technology was the key to ensuring the efficacy and safety of mRNA drugs, which plays an important role in protecting RNA structure, enhancing targeting ability, reducing the dose of drug delivery, and reducing toxic side effects. Lipid nanoparticles (LNP) were the most common delivery system for mRNA drugs. In recent years, mRNA drugs have seen rapid development, with the number of drugs on the market increasing each year. The success of commercializing mRNA vaccines has driven a wave of nucleic acid drug development. mRNA drugs were clinically used in genetic diseases, oncology, and infectious diseases worldwide, while domestic mRNA clinical development was focused on COVID-19 vaccines, with more scope for future indication expansion.
Introduction
Definition and classification of mRNA products
Nucleic acids were the carriers of genetic information for all living organisms and include two major categories: deoxyribonucleic acid (DNA) and ribonucleic acid (RNA) (1). With the development of molecular biology, it has been discovered that in addition to protein-coding nucleic acid sequences, there were also a large number of non-coding sequences that play an important regulatory role in human life activities, such as promoters, enhancers, nucleases, miRNAs, etc (2–6). mRNA products can be divided into mRNA vaccines and mRNA drugs (7, 8) (Figure 1). Drugs that utilized the translational or regulatory functions of mRNA molecules as interventions for diseases are mRNA drugs (9). Therefore, compared to traditional small molecule drugs and antibody drugs, mRNA drugs could intervene at the source by inhibiting the expression of disease-related genes as pathological proteins or introducing genes that can express normal proteins to compensate for the lack of functional proteins, which had the characteristic of “treating the symptoms and the root” (10). In addition, mRNA drugs had the advantages of high therapeutic efficiency, low drug toxicity, and high specificity, and currently had great potential in the treatment of metabolic diseases, genetic diseases, cancer, and prevention of infectious diseases (11).
Principle of mRNA drug therapy
Messenger RNA (mRNA) conveyed genetic information in DNA and synthesizes proteins through translation. mRNA entered the body which was expressed by autologous cells to produce specific proteins, avoiding the influence of in vitro factors; it can regulate the body’s immune system through the endogenous expression of functional proteins and eliminate autologous threats, including cancer cells (12). Compared to conventional therapies, mRNA therapies have a variety of advantages such as being more targeted, simpler to synthesize, more widely adaptable, and alternative to protein therapy (13). An mRNA vaccine encoding an antigen sequence is introduced into cells via a delivery platform such as a lipid nanocarrier and is then translated by human cells to produce the antigen and activate an immune response (14). By endogenously expressing antigenic proteins, mRNA vaccines can induce a more widespread and effective cellular and humoral immune response, resulting in higher protection rates than conventional vaccines (15). Germinal centers are the key to a long-lasting immune response, the place where immune memory is formed, and the longer they are present, the stronger and more durable the immunity will be. Researchers point out that the success of a vaccine depends on the ability to trigger a durable, high-affinity antibody response (16) (Figures 2A, B).
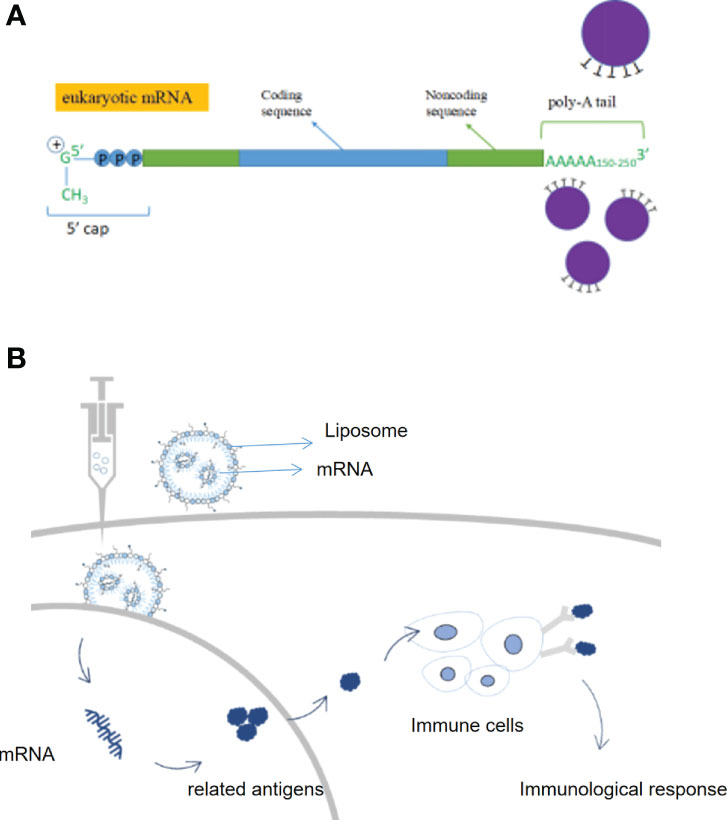
Figure 2 Structure and therapeutic principles of mRNA drugs. (A) Key structures of mRNA products, (B) Mechanism of action of mRNA vaccines.
Not only does mRNA work as a vaccine, but mRNA drug replacement therapy is also one of the main mRNA therapeutic modalities (17). By synthesizing mRNA sequences in vitro and then delivering them into cells by delivery systems, mRNA injected into patients can initiate the production of drugs in the patient’s cells, compensate for defective genes/proteins, transform various protein drugs such as monoclonal antibodies, enzymes, and cytokines in the form of mRNA platforms and be used to treat a wide range of diseases such as metabolic disorders, heart disease, and immuno-oncology (1, 12, 13). Compared to recombinant proteins or small molecules, RNA therapeutics are relatively simple to develop and manufacture and are more cost-effective. In addition, RNA sequences can be easily modified to personalize RNA therapies. mRNA can also be applied in the field of gene editing. mRNA is capable of encoding nucleases like Cas9 in the cytoplasm, with the advantage of rapid expression and no risk of gene integration (5). Cell therapy is one of the main therapeutic applications of mRNA (18). After obtaining cells from a cell bank, they are therapeutically modified in vitro with mRNA encoding the desired protein, and the mRNA-enhanced cells are then reinfused into the patient to treat the disease. Several cell therapies that use mRNA are currently in clinical trials. Examples are TriMix-based immunotherapy (ECI-006), autologous cell therapy CAR-T MCY-M11 (MaxCyte), and Cartesian therapy (19). mRNA has the potential to prevent and treat a wide range of diseases, and as a breakthrough technology platform, is expected to partially replace traditional drugs and vaccines, opening up new therapeutic frontiers and revolutionizing new treatments (20).
Prospects for mRNA therapeutics
In the field of vaccines against infectious diseases, mRNA vaccines have excellent prophylactic potential, can rapidly modify sequences to respond to the emergence of new mutant strains, are effective against immune escape pathogens, and do not integrate into the genome, offering safety advantages (21). In the field of therapeutic cancer vaccines and immuno-oncology vaccines, mRNA vaccines can activate the host’s anti-tumor immunity and also modulate the immunosuppressive tumor microenvironment in solid tumors, thereby inhibiting tumor growth and promising to prolong clinical survival and reduce cancer recurrence rates (22, 23). In the field of protein replacement therapies, the mRNA platform is suitable for most protein-based drugs. It can avoid unnecessary immune responses, has a broader therapeutic scope, and is less difficult to develop than protein-based therapies (24).
mRNA was discovered in 1961, and mRNA received little attention at the time due to its low stability (25). As mRNA has become better understood, it has now become an ideal platform for the treatment of many disease areas (26). mRNA therapy has unique advantages over traditional therapies. Because mRNA is translated into target proteins within the cell, the mRNA-encoded proteins can target both intracellular and cellular membranes, making them more effective than injected proteins, which can only work through extracellular interactions (27). The properties of mRNA make it free from potential infection, insertional mutations, and genomic integration risks. mRNA can be degraded by normal cellular processes, reducing the risk of toxicity. mRNA’s immunogenicity can be down-regulated to further improve safety (28). mRNA vaccines have the potential for rapid and scalable production due to the high yield of the in vitro transcriptional response. The approval and commercialization of mRNA vaccines during the COVID-19 epidemic has increased the scale of global mRNA production and further reduced raw material prices (29). mRNA can be used to prevent and treat different diseases and once this system has been established and validated, only the sequence of the mRNA needs to be changed for use in other products (30). This ensures that development cycles can be significantly shortened and costs can be reduced (31).
History of mRNA therapy development
Since the discovery of mRNA in 1961, the therapeutic potential of mRNA for the disease has been continuously explored (32). In 1987, Robert Malone conducted a landmark experiment in which he mixed mRNA with fat droplets and discovered that this mRNA could be taken up by frog embryo cells, a discovery that laid the foundation for the idea that RNA could be considered a drug delivered into the body to express (33). Since the 1970s, delivery systems have been optimized and updated, and there has been increasing interest in the immune response to mRNA once it enters the body (34). It was found that nucleoside modifications could reduce the immunogenicity of mRNAs and allow them to be accepted by the body for the treatment of disease (35). As the ability of mRNA to treat disease is explored, different mRNA therapies are emerging, including alternative therapies, mRNA vaccines, immunotherapies, gene editing therapies, etc (36–38). Pre-clinical human trials have been conducted for different therapies, and disease-specific mRNA vaccines and proven delivery systems have been developed, which may be developed on a large scale for disease prevention and treatment in the future (Figure 3). mRNA therapies break through many of the limitations of traditional therapies and have the potential to become a novel therapeutic alternative to traditional treatments (39, 40).
Current mRNA drug development
Now, there are two mRNA products on the market globally(Table 1). In December 2020, the FDA approved two new mRNA COVID-19 vaccines from Pfizer with BioNTech and Moderna for emergency use, with formal approval for both products in 2021 and 2022, respectively (41, 42).
The mRNA drugs currently under development can be divided into three main categories by use and drug type: prophylactic vaccines, therapeutic vaccines, and therapeutic drugs (43–45). According to statistics, there are 56 mRNA drugs in the clinical pipeline worldwide, with R&D mainly focused on vaccines, accounting for about 84%, while therapeutic drugs account for about 16%. Except for the mRNA COVID-19 vaccine, which is urgently marketed, most others are in the early stages. mRNA R&D is mainly in clinical phase I, accounting for about 40% of the total number (46).
The range of applications for mRNA is extremely broad. The main applications for mRNA currently include three major directions: immunotherapy, protein replacement therapy, and regenerative medicine therapy (47–49). Among the immunotherapies, tumor immunotherapy and infectious vaccines are the most popular and mature, and the mRNA pipeline under development mainly targets genetic diseases such as infectious diseases, oncological diseases, and rare diseases. For delivery systems, the commonly used carriers for mRNA include viral-based delivery systems, fisetin, and lipid nanoparticles, among which lipid nanoparticle delivery systems are the most commonly used carriers, accounting for about 81% of the total (50). mRNA drug development’s main technical threshold lies in stability and delivery, and the delivery system is crucial to the effect of mRNA. The rapid responsiveness, universal adaptability, and rapid production capacity of mRNA-based drugs make them highly promising for use in a wide range of diseases (51).
Since the outbreak of the COVID-19 epidemic, domestic listed pharmaceutical companies have started a boom in collaborating with mRNA companies to develop vaccines. In terms of COVID-19 vaccines, domestic Watson/Aibo/ARCoV from the Academy of Military Sciences is in clinical phase III (52), BNT162b2 from Fosun Pharma and BioNTech is in clinical phase II (53). The domestic vaccine industry is expected to compete with overseas markets at the same stage; most of the other indications are in the pre-clinical/early stage. At present, the domestic mRNA research field is dominated by COVID-19 vaccines, while global mRNA drug indications are distributed in genetic diseases, COVID-19, and other infectious diseases, compared to the future indications of domestic mRNA drugs, there is more room for expansion (54).
The year 2020 was a breakthrough year for mRNA, with two mRNA COVID-19 vaccines developed by Pfizer BioNTech and Moderna, respectively, receiving emergency use authorizations. mRNA COVID-19 vaccines will generate global sales of US$58.7 billion in 2021, and both far exceed sales of other COVID-19 vaccines (55, 56). mRNA technology can be used primarily in vaccines, immunotherapies, and protein replacement therapies. In the case of COVID-19 vaccines, mRNA vaccines offer advantages in terms of development efficiency and protection rates. In the short term, the market for preventive vaccines will be dominated by COVID-19 products, while in the medium to long term, mRNA vaccines will expand into the prevention of more infectious diseases, such as influenza virus vaccines, influenza respiratory syncytial virus vaccines, and malaria vaccines, due to their advantages in terms of the number of targets, efficacy, safety and manufacturing processes (57, 58). In addition to preventive vaccines, immunotherapy for tumors is also a hot topic of research in mRNA technology (48). Traditional immunotherapy requires the injection of antigens into the body, which is difficult and expensive to synthesize. In the field of oncology treatment, there is a large number of patients and unmet clinical needs, and the market size for therapeutic oncology vaccines will continue to climb in the future (Figure 4). Overall, with improvements and advances in delivery technology and stability, mRNA has a very strong competitive advantage and has the potential to become a cross-application product (59).
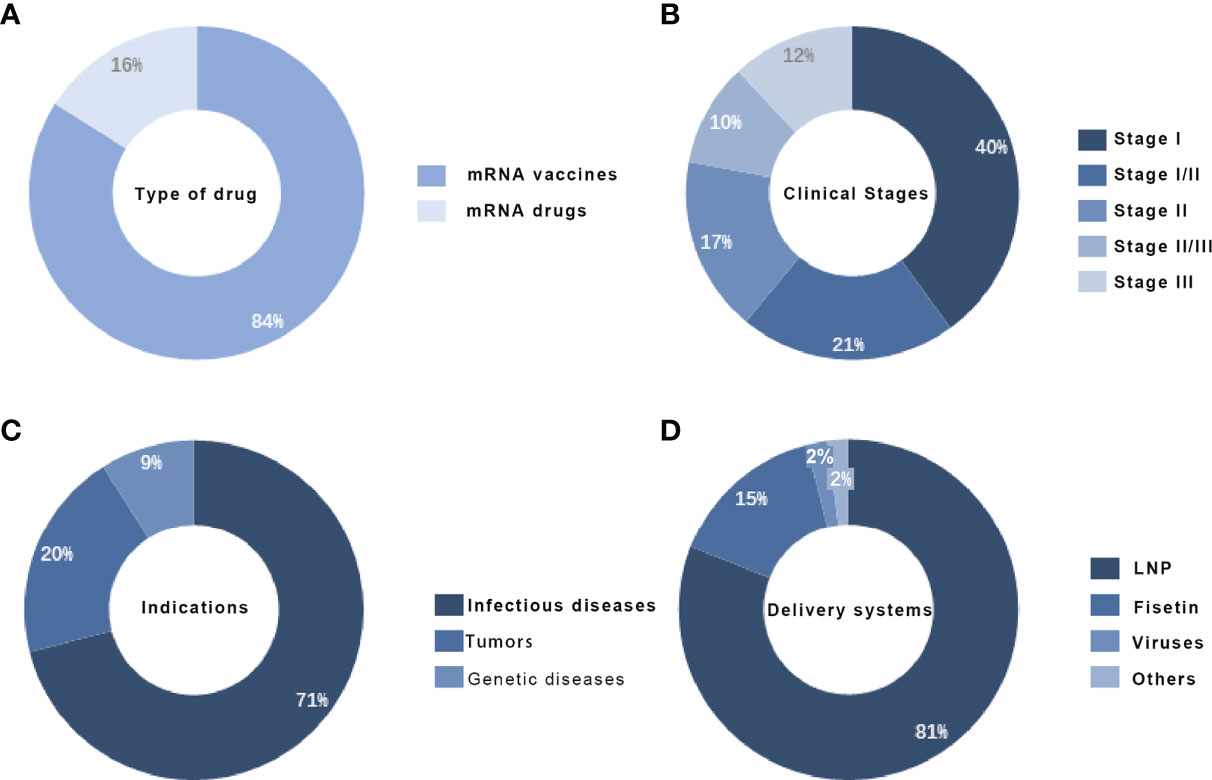
Figure 4 Global clinical mRNA distribution, (A) Global mRNA product type distribution (B) Global mRNA drug clinical staging distribution (C) Global mRNA drug indication distribution(D) Global mRNA drug delivery system distribution.
mRNA drug key development technologies and challenges
Key issues and challenges in mRNA product development
According to the central law, mRNA theoretically had the potential to be expressed as any kind of protein through the ribosome and therefore can be used to treat a wide range of diseases, and mRNA is cheaper to produce than recombinant protein drugs (60). However, mRNA technology still needs to address the pain points of immunogenicity, efficiency in expressing proteins in vivo, and ultimately scale up production, so innovations in key development technologies such as sequence design, synthetic design of LNP delivery systems, and optimization of manufacturing processes could facilitate the development of mRNA drugs (61). mRNA design sequence optimization - both coding and non-coding sequences can be optimized (62). The translation efficiency of mRNAs can be improved by optimizing the coding sequence (63). Optimizing non-coding sequences can also increase translation efficiency and mRNA stability(2). However, the process of sequence optimization requires specific skills and experience. The immune system can recognize unmodified single-stranded RNA, causing a decrease in protein expression and the development of reactogenicity (64). Translation efficiency can be improved by introducing modified nucleotides, the most commonly used modified nucleoside, N1-methyl-pseudouridine, requires a patent license (65). As mRNA is a negatively charged biomolecule, it is difficult to pass through the negatively charged lipid bilayer on the surface of cell membranes (66). In addition, mRNA is susceptible to phagocytosis by immune system cells and degradation by nucleases, as well as having intracellular release challenges. Therefore, an efficient and safe delivery system is essential for mRNA drugs. LNP is currently the most clinically advanced mRNA delivery vehicle and is highly feasible and patentable (67). However, its synthetic design needs to be optimized to address its toxicity and tendency to aggregate and leak. The purity of mRNA has a significant impact on efficacy and safety, and efficient purification methods need to be developed to improve mRNA purity and remove impurities such as double-stranded RNA, truncated mRNA, and DNA residues (68). mRNA particle size uniformity can improve the stability of the LNP delivery system (69). In large-scale production, specific encapsulation techniques can improve encapsulation efficiency, ensure consistent quality and save production costs (70).
mRNA development technologies
The core technical challenges at the design and synthesis level are mastering platform-based computational capabilities, and 5’ end-capping and UTR region nucleotide modifications are key points (71). At the modification level, chemical modifications improve drug stability and reduce toxicity (72). At the level of delivery system synthesis and design, the synthesis of lipid nanoparticles mRNA-LNP is a key focus of R&D in the mRNA field (20). mRNA vaccine or drug scale-up production is highly reproducible, with successful sequence modification and delivery system assembly at the core. The upstream and downstream chains of scale-up production are also particularly important, involving hundreds of enzymes, nucleotides, liposomes, and other raw materials, for example, and presenting many technical challenges such as the difficulty of mass production of raw materials and high barriers to production equipment (Table 2).
For the new mRNA COVID-19 vaccines already licensed, accessibility was further limited by refrigeration conditions. If billions of people are to be vaccinated globally, a vaccine with better heat resistance is required. In preclinical studies, CureVac has demonstrated that the sequence-optimized rabies virus vaccine in development, RABV-G, could remain stable for several months in the region between -80°C and 70°C (73). Furthermore, two investigational mRNA COVID-19 vaccines had now been reported to be able to remain stable in a room temperature environment (74, 75). If these heat-resistant vaccine candidates achieve positive results in clinical trials, it is expected to improve the global accessibility of mRNA vaccines (Table 3).
Technical analysis of mRNA drug delivery systems
The development of mRNA delivery systems has gone through the stage of fisetin, a naturally occurring cationic protein that can complex negatively charged mRNA molecules into nano-sized nucleic acid particles to protect mRNA from degradation by RNA enzymes in serum, which was discovered in 1961 (32). Cationic polymers neutralize the negative charge of the nucleic acid drug to increase the efficiency of entry into the cell (76). Polyethyleneimine (PEI) was a common polymer used for gene delivery, but the high molecular weight of PEI resulted in high cytotoxicity, making its successful use for vaccines in a limited number of animal studies, and chemical modifications of PEI were continually being explored (77). Liposomes began to be used as a carrier-optimized route of administration in 1978, and cationic lipids were first proposed to mediate mRNA transcription in 1989, until the successful launch of mRNA COVID-19 vaccines in 2020 (78–80). Lipids are currently the most widely used class of delivery systems for nucleic acid drugs, including liposomes and lipid nanoparticles, the most commonly used being LNPs containing ionizable lipids (Figure 5). The first generation was 1,2-dioleylidene-3-dimethylaminopropane (DLinDMA) and the second generation included DLin-MC3-DMA, which was further optimized based on the first generation and led to the development of drugs such as Patisiran and ALN-PCS (81). In contrast to the first generation, DLin-MC3-DMA has a unique pH-dependent charge-variable property: it is positively charged under acidic conditions and electrically neutral under physiological pH conditions (82). It protonates in the acidic environment of endosomes or lysosomes, promoting membrane fusion of LNP with endosomes and allowing mRNA to escape from endosomes/lysosomes to function (51) (Table 4).
The advantages of using an LNP delivery system include the following: the use of LNP removes the risk of infection, oncogenicity, and immunogenicity compared to viral vectors (83); LNP can be introduced into the target cell by decorating the cell surface with ligands; LNP prevents mRNA degradation and aids endocytosis and endosomal escape, thus enhancing antigen expression and the efficiency of mRNA vaccination; adjuvants can be added to LNP to aid immune activation and potential immune response (20).
The side effects of mRNA vaccines
Regarding mRNA side effects, there had been reported high rates of vaccination-related side effects, including pericarditis, myocarditis, neurological inflammation, and autoimmune hepatitis (84–87). Although published data from clinical study sponsors and others suggested that these side effects are not related to the vaccine itself (88). mRNA vaccine side effects occur at a rate of 80-90%, with moderate to severe side effects reaching around 10%, although the majority are common low-grade side effects (89). Studies from academic institutions showed that the majority of side effects are due to LNP components such as PEG and ionized lipids, with both natural and adaptive immunity involved. To improve vaccine efficacy and limit side effects, researchers were changing the four components that make up the lipid nanoparticles. Each particle contains ionizable lipids that bind messenger ribonucleic acid (mRNA), and once inside the body, their charge shifts from positive to neutral to limit the toxicity of the particles. The other three lipids contribute to their structure and stability. Auxiliary lipids also help the particles fuse with the cell, cholesterol helps them escape the cell’s endosome, and polyethylene glycol (PEG) liposomes prevent them from aggregating, thus prolonging their action. Dan Peer has also developed novel ionized lipid libraries with atypical structures (90, 91). In unpublished experiments, they appear to enable better mRNA vaccines with fewer side effects and prolong their stability at room temperature.
Other improvements might come from facilitating the uptake of LNPs into the cell and then enhancing their ability to break free of the cell membrane vesicles, known as endosomes, that carried them into the cell (92). The vast majority of LNPs are trapped in these vessels and then destroyed or expelled without delivery of the vaccine payload, meaning that a significant amount of RNA is not used. The shape of ionizable lipids affects the ability of LNPs to destroy endosomes, and cholesterol is another lipid in LNPs (93). Sahay reports that the use of different forms of cholesterol can improve the escape rate of LNPs, and Sanofi has begun to evaluate its bespoke LNPs positively in human trials (94). which announced that a lipid formulation proved to be more effective in initiating anti-flu immunity. Other companies, including BioNTech and Arcturus Therapeutics, had begun exploring the elimination of polyethylene glycol, a compound that helps stabilize LNPs but is also associated with certain types of adverse vaccine reactions (95). At the same time, more and more companies were focusing on optimizing lipids to deliver mRNA to treat disease, rather than prevent it. This requires access to mRNAs that can encode disease-correcting proteins into the precise cells and tissues, not just the liver, as current preparations of LNPs tend to end up after infusion. Delivery of LNPs will be key to truly expanding the reach of mRNA beyond prophylactic vaccines.
mRNA drug industry chain and development trend
The mRNA drug industry chain
The mRNA drug industry chain covers the upstream preparation of raw materials, the midstream development and production of biopharmaceutical companies, and the downstream commercialization of products for patients(Figure 6). mRNA technology faces challenges in sequence optimization and synthesis, delivery systems, and large-scale formulation and storage (96). Continuous optimization of technology will continue to drive the rapid expansion of the mRNA market. In terms of mRNA synthesis and sequence optimization, sequence optimization is required to improve the stability and expression efficiency of mRNA due to its relatively short half-life, and further sequence improvement is required to control immunogenicity as mRNA can induce intrinsic immunogenicity (97). mRNA optimization directions include: 5’ cap addition, 5’ untranslated region (5’UTR), open reading frame (ORF) region, 3’ untranslated region (3’UTR) and polyA tail structure (72). In terms of delivery systems, liposomal nanoparticles are currently the preferred mRNA delivery system, which is virtually non-toxic to most cells, but can still have side effects on the liver in some cases, and future delivery systems need to be further optimized (98). In terms of large-scale preparation and storage, although the mRNA production process is not complex, it is relatively early in the field and a stable and controlled supply chain for large-scale production has not yet been established. mRNA products require ultra-low temperature transport and storage, breakthroughs in storage methods will facilitate their rapid development.
To date, clinical studies had demonstrated that siRNA drugs can be delivered effectively in vivo using LNPs, and with the approval of the LNP-mediated siRNA therapeutic agent Onpattro® (patisiran), the use of LNPs for delivery was one of the first ideas in the field of mRNA delivery (99). Two LNPs, mRNA-1273 and BNT162b2, had now been used in clinical trials. In recent years, LNPs had also shown great potential for use in self-amplifying mRNA vaccines and conventional non-replicating mRNAs. mRNA-LNP complexes delivered by LNPs systems were primarily targeted at the liver, however, the mechanism of mRNA escaping into the cytoplasm was not fully understood. New directions for the continued development of LNPs systems were focused on ionizable lipids and formulations, all of which combinations of optimization areas are almost limitless, and success in any of these parameters could beneficial effects and provide new approaches to successful vaccine disease prevention (76).
However, there were still problems to be overcome in the delivery of mRNA vaccines, such as the toxicity of some lipid formulations and the difficulty of reaching immune cells in specialized secondary lymphoid organs. Multi-antigen vaccines were also a major challenge in mRNA vaccine development, for example, the number of LNP:mRNA ratios are typically around 10:1 to 30:1, and multi-antigen candidates require large numbers of LNPs, which have inherent adjuvant properties, so safety and tolerability may limit the development of multi-antigen mRNA vaccines (100).
With the continuous maturation of mRNA in vitro synthesis and delivery technology in recent years, the stability and translation efficiency of mRNA drugs have improved significantly, and mRNA technology has been able to develop rapidly (101). Also driven by the emergence of a large number of biotechnology companies and a boom in capital market investment and financing, the future mRNA drug market will show the following trends: Expansion of therapeutic areas, mRNA is a revolutionary platform for the production of antigens or drugs using cells and is suitable for most vaccine products. There are proven mRNA vaccine platforms that manufacturers can apply to other diseases by simply changing the mRNA sequence (102). As a result, mRNA-based therapies can be rapidly scaled up to a wide range of other diseases. mRNA therapies are also potential alternatives to many future protein-based drugs such as cytokines and even antibodies, and mRNA-based therapies have the potential to be further extended to many other disease treatments (103). In addition, mRNA drug development could also target some of the targets that are now difficult to drug, further expanding the therapeutic field. The rapid development of the upstream and downstream of the industry chain: The outbreak of the new COVID-19 epidemic in 2020 has accelerated the popularity and commercialization of mRNA technology, with countries increasing their investment in mRNA technology and giving rise to the birth of mRNA biotechnology companies, promoting the construction and improvement of mRNA and drug technology platforms, while bringing about an explosion of opportunities upstream and downstream of the mRNA industry chain, driving the development of mRNA drugs, including upstream raw materials, midstream research and development and manufacturing, and downstream drug discovery (104). R&D and manufacturing, as well as downstream distribution and transportation. Improved efficacy and safety: Compared with traditional vaccines, mRNA vaccines have higher efficacy but also have relatively greater side effects. mRNA drug efficacy and safety control are also critical to clinical practice. As mRNA research progresses and new technologies such as AIs are developed, better mRNA codon optimization, the use of modified bases, and improvements in delivery systems can produce higher immune responses at lower doses. The use of new mRNA platform technologies, such as self-amplifying mRNA, is also a potential approach to reduce the dose and ensure clinical effectiveness while reducing side effects (105). Gradual completion of R&D system: Currently, mRNA pharmaceutical companies have formed complete mRNA vaccine teams, optimized mRNA high-throughput synthesis platforms, improved patent systems, enriched mRNA pipelines, and advanced processes, developed leading mRNA delivery technologies, and gradually scaled up to mass production technologies (106). In the future, the mRNA industry will make breakthroughs in drug development technology and gradually improve in all aspects such as product lines, formulation technology, patent layout, and production processes (107). Cooperation in commercialization, after the start of the epidemic, many COVID-19 vaccine companies in various countries responded quickly and started rapid research and development of COVID-19 vaccines, actively introducing leading products and reaching cooperation with relevant companies to obtain commercialization rights of mRNA vaccines (108). At the same time, the different experiences and core competencies of national companies in mRNA chemical modification, targeted nucleic acid drug delivery formulation, vaccine registration, clinical research, industrialization, and marketing have led to multiple collaborations between companies.
There was a growing interest in developing combination vaccines to protect against more than one disease using a single vaccine. Non-replicating mRNAs encoded only the target antigen, while self-amplifying mRNAs vaccines also encoded the replication mechanism of the virus. The combination of CAR T-cell therapy and mRNA vaccine technology avoids some of the potential problems associated with CAR T-cell therapy in the treatment of hematological malignancies. These include the use of viral vectors that alter cellular DNA (109); T cells need to be harvested from the patient, cultured, and then reintroduced into the patient; the number of T cells that had not been genetically engineered must be reduced by chemotherapy (110). These combination therapies also extend the potential of mRNA technology beyond vaccines. Lin Jinzhong’s group at Fudan University has developed a new crown mRNA vaccine, RQ3013, capable of broadly neutralizing mutant strains of VOCs, encoding a Spike protein carrying the Alpha/Beta/Omicron Spike mutation. In mouse and NHP rhesus monkey models, it strongly triggers high titers of seronegative antibodies targeting the original strains, Alpha (B.1.1.7), Beta (B.1.351), Delta (B.1.627.2), and Omicron (B.1.529). The systematic evaluation of the immunogenicity, immunoprotective efficiency, and safety of RQ3013 in animal models confirmed that it was a promising broad-spectrum vaccine capable of targeting new crown VOCs (111).
In summary, the stability and delivery efficiency of mRNA drugs has been a constraint to drug success. As mRNA is by nature a transient molecule with a short half-life, it is easily degraded, and its large molecular weight and high negative charge density affect its permeability through cell membranes. The specific delivery of mRNA drugs to cells is therefore one of the key bottlenecks in the role of mRNA technology. As the core of mRNA drugs is specificity and stable delivery. The ideal delivery vehicle must be free from enzymatic degradation, be specifically taken up by the target cell, and finally be released from the endosome in time after entry into the cytoplasm. This ensures the stability and translation efficiency of mRNA drugs but is also a hurdle that must be overcome for mRNA drugs to reach the clinical application. This has been achieved by optimizing mRNA structural chemistry and delivery systems. To improve delivery efficiency and minimize therapeutic side effects, a variety of delivery vehicles have been developed to encapsulate mRNA, using natural or synthetic materials (e.g. lipids, polymers, etc.) to make nanoparticles with different geometrical structures (nanoparticles, particles, conjugates, etc.) to safely deliver mRNA to target cells to express normal proteins. Among these, lipid nanoparticles (LNPs) have shown potential for efficient delivery as the only delivery system in clinical validation. At the same time, however, the ability of LNPs to target organs other than the liver has been an insurmountable challenge for the industry and has created an invisible ceiling in the mRNA drug development market. When systemically delivered, can LNPs selectively target specific organs, or even further target specific cells in those organs? This metric is the ultimate goal that the entire delivery technology is intended to achieve.
Conclusion
The introduction of COVID-19 vaccines and the emergence of mRNA companies all point to the fact that mRNA technology is destined to become a spark that can start a new fire, and the widening field of therapeutics and the continued disclosure of data on new therapeutics further demonstrate its endless potential. While the two core technologies of mRNA design and delivery systems are critical to mRNA technology companies, AI technology for R&D platforms is now a strong, if not indispensable, barrier for companies (112). We expect more innovative drug companies to bring together cross-industry and cross-discipline strengths into one, and to overcome the obstacles to bringing innovation to China and the world.
mRNA is one of the most clinically promising frontiers of future biopharmaceuticals, and after years of development, heavyweight products have emerged, showing unprecedented applications in the treatment of metabolic diseases and the prevention of infectious diseases (113). The COVID-19 epidemic has brought mRNA vaccines to widespread public attention and brought about the rapid development of mRNA products, which are also becoming a focus of biopharmaceutical investment and a hotspot for pharmaceutical companies’ research and development. We hope that more people will use this review to understand the opportunities and challenges facing mRNA drugs.
Author contributions
Conceptualization, QD, TH, QZ, XJ, FC, and XC. Investigation, QD, TH, QZ, and XJ. Writing-original draft preparation, QD, FC, and XC. Writing-review and editing, TH, QZ, XJ, and FC. Funding acquisition, XC. All authors have read and agreed to the published version of the manuscript.
Acknowledgments
This review is thanks to Wu Jieping Medical Foundation Clinical Research Grant(320.6750.19093-13).
Conflict of interest
The authors declare that the research was conducted in the absence of any commercial or financial relationships that could be construed as a potential conflict of interest.
Publisher’s note
All claims expressed in this article are solely those of the authors and do not necessarily represent those of their affiliated organizations, or those of the publisher, the editors and the reviewers. Any product that may be evaluated in this article, or claim that may be made by its manufacturer, is not guaranteed or endorsed by the publisher.
References
1. Singh RP, Srivastava AK, Yang YJ, Manchanda G, Kumar A, Yerpude ST, et al. Nucleic acid nanotechnology: Trends, opportunities and challenges. Curr Pharm Biotechnol (2022) doi: 10.2174/1389201023666220520103325
2. Lee SH, Brianna. Therapeutic targeting of overexpressed mirnas in cancer progression. Curr Drug Targets (2022) published online ahead of print, 2022 Jun 13. doi: 10.2174/1389450123666220613163906
3. Tomita N, Tomita T, Yuyama K, Tougan T, Tajima T, Ogihara T, et al. Development of novel decoy oligonucleotides: Advantages of circular dumb-bell decoy. Curr Opin Mol Ther (2003) 5(2):107–12.
4. Sabo PJ, Humbert R, Hawrylycz M, Wallace JC, Dorschner MO, McArthur M, et al. Genome-wide identification of dnasei hypersensitive sites using active chromatin sequence libraries. Proc Natl Acad Sci USA (2004) 101(13):4537–42. doi: 10.1073/pnas.0400678101
5. Lazzarotto CR, Malinin NL, Li Y, Zhang R, Yang Y, Lee G, et al. Change-seq reveals genetic and epigenetic effects on crispr-Cas9 genome-wide activity. Nat Biotechnol (2020) 38(11):1317–27. doi: 10.1038/s41587-020-0555-7
6. Baek S, Sung MH. Genome-scale analysis of cell-specific regulatory codes using nuclear enzymes. Methods Mol Biol (2016) 1418:225–40. doi: 10.1007/978-1-4939-3578-9_12
7. Zimmerman O, Altman Doss AM, Kaplonek P, Liang CY, VanBlargan LA, Chen RE, et al. Mrna vaccine boosting enhances antibody responses against sars-Cov-2 omicron variant in individuals with antibody deficiency syndromes. Cell Rep Med (2022) 3(6):100653. doi: 10.1016/j.xcrm.2022.100653
8. Qu G, Chen J, Li Y, Yuan Y, Liang R, Li B. Current status and perspectives of regulatory T cell-based therapy. J Genet Genomics = Yi Chuan xue bao (2022) 49(7):599–611. doi: 10.1016/j.jgg.2022.05.005
9. Zhao J, Li Z, Puri R, Liu K, Nunez I, Chen L, et al. Molecular profiling of individual fda-approved clinical drugs identifies modulators of nonsense-mediated mrna decay. Mol Ther Nucleic Acids (2022) 27:304–18. doi: 10.1016/j.omtn.2021.12.003
10. Sahin U, Kariko K, Tureci O. Mrna-based therapeutics–developing a new class of drugs. Nat Rev Drug Discovery (2014) 13(10):759–80. doi: 10.1038/nrd4278
11. Suzuki Y, Ishihara H. Difference in the lipid nanoparticle technology employed in three approved sirna (Patisiran) and mrna (Covid-19 vaccine) drugs. Drug Metab Pharmacokinet (2021) 41:100424. doi: 10.1016/j.dmpk.2021.100424
12. Dai H, Abdullah R, Wu X, Li F, Ma Y, Lu A, et al. Pancreatic cancer: Nucleic acid drug discovery and targeted therapy. Front Cell Dev Biol (2022) 10:855474. doi: 10.3389/fcell.2022.855474
13. Wu J, Cao J, Fan Y, Li C, Hu X. Comprehensive analysis of mirna-mrna regulatory network and potential drugs in chronic chagasic cardiomyopathy across human and mouse. BMC Med Genomics (2021) 14(1):283. doi: 10.1186/s12920-021-01134-3
14. Corey KB, Koo G, Stone CA Jr., Kroop SF, Fissell WH, Kozlowski S, et al. A case of coronavirus disease 2019 messenger rna vaccine tolerance and immune response despite presence of anti-polyethylene glycol antibodies. Ann allergy Asthma immunol: Off Publ Am Coll Allergy Asthma Immunol (2022) 129(2):246–248. doi: 10.1016/j.anai.2022.05.013
15. Fava VM, Bourgey M, Nawarathna PM, Orlova M, Cassart P, Vinh DC, et al. A systems biology approach identifies candidate drugs to reduce mortality in severely ill patients with covid-19. Sci Adv (2022) 8(22):eabm2510. doi: 10.1126/sciadv.abm2510
16. Turner JS, O’Halloran JA, Kalaidina E, Kim W, Schmitz AJ, Zhou JQ, et al. Sars-Cov-2 mrna vaccines induce persistent human germinal centre responses. Nature (2021) 596(7870):109–13. doi: 10.1038/s41586-021-03738-2
17. Takeuchi T, Okuno Y, Hattori-Kato M, Zaitsu M, Mikami K. Detection of ar-V7 mrna in whole blood may not predict the effectiveness of novel endocrine drugs for castration-resistant prostate cancer. Res Rep Urol (2016) 8:21–5. doi: 10.2147/RRU.S98877
18. Haist M, Mailander V, Bros M. Nanodrugs targeting T cells in tumor therapy. Front Immunol (2022) 13:912594. doi: 10.3389/fimmu.2022.912594
19. Fernandez AMA, Baurain JF, Vulsteke C, Rutten A, Soria A, Carrasco J, et al. A phase I study (E011-Mel) of a trimix-based mrna immunotherapy (Eci-006) in resected melanoma patients: Analysis of safety and immunogenicity. J Clin Oncol (2019) 37(15):S2645. doi: 10.1200/JCO.2019.37.15_suppl.2641
20. Kiaie SH, Majidi Zolbanin N, Ahmadi A, Bagherifar R, Valizadeh H, Kashanchi F, et al. Recent advances in mrna-lnp therapeutics: Immunological and pharmacological aspects. J nanobiotechnol (2022) 20(1):276. doi: 10.1186/s12951-022-01478-7
21. Tenforde MW, Self WH, Zhu Y, Naioti EA, Gaglani M, Ginde AA, et al. Protection of mrna vaccines against hospitalized covid-19 in adults over the first year following authorization in the united states. Clin Infect Dis (2022). doi: 10.1093/cid/ciac381
22. Tan H, Yu T, Liu C, Wang Y, Jing F, Ding Z, et al. Identifying tumor antigens and immuno-subtyping in colon adenocarcinoma to facilitate the development of mrna vaccine. Cancer Med (2022). doi: 10.1002/cam4.4846
23. Suthar MS, Arunachalam PS, Hu M, Reis N, Trisal M, Raeber O, et al. Durability of immune responses to the Bnt162b2 mrna vaccine. Med (2022) 3(1):25–7. doi: 10.1016/j.medj.2021.12.005
24. Biggs AT, Littlejohn LF. Describing mrna vaccine technology for a military audience. Military Med (2022). doi: 10.1093/milmed/usac129
25. Shommo G, Apolloni B. A holistic mirna-mrna module discovery. Non-coding RNA Res (2021) 6(4):159–66. doi: 10.1016/j.ncrna.2021.09.001
26. Di Trani CA, Fernandez-Sendin M, Cirella A, Segues A, Olivera I, Bolanos E, et al. Advances in mrna-based drug discovery in cancer immunotherapy. Expert Opin Drug Discovery (2022) 17(1):41–53. doi: 10.1080/17460441.2021.1978972
27. Ichihashi Y, Fukushima A, Shibata A, Shirasu K. High impact gene discovery: Simple strand-specific mrna library construction and differential regulatory analysis based on gene Co-expression network. Methods Mol Biol (2018) 1830:163–89. doi: 10.1007/978-1-4939-8657-6_11
28. Zeng G. Safety and immunogenicity of the sars-Cov-2 arcov mrna vaccine. Lancet Microbe (2022) 3(8):e561. doi: 10.1016/S2666-5247(22)00150-1
29. Coats MT, Bydlinski N, Maresch D, Diendorfer A, Klanert G, Borth N. Mrna transfection into cho-cells reveals production bottlenecks. Biotechnol J (2020) 15(2):e1900198. doi: 10.1002/biot.201900198
30. Wommer L, Meiers P, Kockler I, Ulber R, Kampeis P. Development of a 3d-printed single-use separation chamber for use in mrna-based vaccine production with magnetic microparticles. Eng Life Sci (2021) 21(10):573–88. doi: 10.1002/elsc.202000120
31. Shepherd SJ, Warzecha CC, Yadavali S, El-Mayta R, Alameh MG, Wang L, et al. Scalable mrna and sirna lipid nanoparticle production using a parallelized microfluidic device. Nano Lett (2021) 21(13):5671–80. doi: 10.1021/acs.nanolett.1c01353
32. Gros F, Gilbert W, Hiatt HH, Attardi G, Spahr PF, Watson JD. Molecular and biological characterization of messenger rna. Cold Spring Harbor Symp quantitat Biol (1961) 26:111–32. doi: 10.1101/sqb.1961.026.01.016
33. Malone RW, Felgner PL, Verma IM. Cationic liposome-mediated rna transfection. Proc Natl Acad Sci USA (1989) 86(16):6077–81. doi: 10.1073/pnas.86.16.6077
34. Clissold PM, Arnstein HR, Chesterton CJ. Quantitation of globin mrna levels during erythroid development in the rabbit and discovery of a new beta-related species in immature erythroblasts. Cell (1977) 11(2):353–61. doi: 10.1016/0092-8674(77)90052-6
35. Ni Z, Sun P, Zheng J, Wu M, Yang C, Cheng M, et al. Jnk signaling promotes bladder cancer immune escape by regulating Mettl3-mediated M6a modification of pd-L1 mrna. Cancer Res (2022) 82(9):1789–802. doi: 10.1158/0008-5472.CAN-21-1323
36. Ponger P, Kurolap A, Lerer I, Dagan J, Chai Gadot C, Mory A, et al. Unique ataxia-oculomotor apraxia 2 (Aoa2) in Israel with novel variants, atypical late presentation, and possible identification of a poison exon. J Mol neuro: MN (2022) 72(8):1715–1723. doi: 10.1007/s12031-022-02035-5
37. Imbalzano G, Ledda C, Artusi CA, Romagnolo A, Montanaro E, Rizzone MG, et al. Sars-Cov-2 vaccination, parkinson’s disease, and other movement disorders: Case series and short literature review. Neurol sciences: Off J Ital Neurol Soc Ital Soc Clin Neurophysiol (2022) 43(9):5165–5168. doi: 10.1007/s10072-022-06182-w
38. Chemaitelly H, Ayoub HH, AlMukdad S, Coyle P, Tang P, Yassine HM, et al. Duration of mrna vaccine protection against sars-Cov-2 omicron Ba.1 and Ba.2 subvariants in Qatar. Nat Commun (2022) 13(1):3082. doi: 10.1038/s41467-022-30895-3
39. Zabaleta N, Torella L, Weber ND, Gonzalez-Aseguinolaza G. Mrna and gene editing: Late breaking therapies in liver diseases. Hepatology (2022) 76(3):869–887. doi: 10.1002/hep.32441
40. Capone F, Lucchini M, Ferraro E, Bianco A, Rossi M, Cicia A, et al. Immunogenicity and safety of mrna covid-19 vaccines in people with multiple sclerosis treated with different disease-modifying therapies. Neurotherapeutics (2022) 19(1):325–33. doi: 10.1007/s13311-021-01165-9
41. Booster dose of the Pfizer/Biontech covid-19 vaccine (Comirnaty). Med letter Drugs Ther (2021) 63(1635):161–2.
42. Covid-19 updates: Moderna covid-19 vaccine (Spikevax) gains full licensure. Med letter Drugs Ther (2022) 64(1644):32.
43. Weng Y, Huang Y. Advances of mrna vaccines for covid-19: A new prophylactic revolution begins. Asian J Pharm Sci (2021) 16(3):263–4. doi: 10.1016/j.ajps.2021.02.005
44. Guevara ML, Persano S, Persano F. Lipid-based vectors for therapeutic mrna-based anti-cancer vaccines. Curr Pharm design (2019) 25(13):1443–54. doi: 10.2174/1381612825666190619150221
45. Bidram M, Zhao Y, Shebardina NG, Baldin AV, Bazhin AV, Ganjalikhany MR, et al. Mrna-based cancer vaccines: A therapeutic strategy for the treatment of melanoma patients. Vaccines (2021) 9(10):1060. doi: 10.3390/vaccines9101060
46. DiGregorio BD, Corcoran KE, Scheitle CP. ‘God will protect us’: Belief in God/Higher power’s ability to intervene and covid-19 vaccine uptake. Rev religious Res (2022) published online ahead of print, 2022 Jun 9:1–21. doi: 10.1007/s13644-022-00495-0
47. Kwon S, Kwon M, Im S, Lee K, Lee H. Mrna vaccines: The most recent clinical applications of synthetic mrna. Arch pharmacal Res (2022) 45(4):245–62. doi: 10.1007/s12272-022-01381-7
48. Bhat B, Karve S, Anderson DG. Mrna therapeutics: Beyond vaccine applications. Trends Mol Med (2021) 27(9):923–4. doi: 10.1016/j.molmed.2021.05.004
49. Xiao Y, Tang Z, Huang X, Chen W, Zhou J, Liu H, et al. Emerging mrna technologies: Delivery strategies and biomedical applications. Chem Soc Rev (2022) 51(10):3828–45. doi: 10.1039/d1cs00617g
50. Tsiambas E, Chrysovergis A, Papanikolaou V, Mastronikolis N, Ragos V, Batistatou A, et al. Impact of ribosome activity on sars-Cov-2 lnp - based mrna vaccines. Front Mol Biosci (2021) 8:654866. doi: 10.3389/fmolb.2021.654866
51. Ndeupen S, Qin Z, Jacobsen S, Bouteau A, Estanbouli H, Igyarto BZ. The mrna-lnp platform’s lipid nanoparticle component used in preclinical vaccine studies is highly inflammatory. iScience (2021) 24(12):103479. doi: 10.1016/j.isci.2021.103479
52. Chen GL, Li XF, Dai XH, Li N, Cheng ML, Huang Z, et al. Safety and immunogenicity of the sars-Cov-2 arcov mrna vaccine in Chinese adults: A randomised, double-blind, placebo-controlled, phase 1 trial. Lancet Microbe (2022) 3(3):E193–202. doi: 10.1016/S2666-5247(21)00280-9
53. Goldin S, Adler L, Azuri J, Mendel L, Haviv S, Maimon N. Bnt162b2 mrna covid-19 (Comirnaty) vaccine effectiveness in elderly patients who live in long-term care facilities: A nationwide cohort. Gerontology (2022), 1–8 published online ahead of print. doi: 10.1159/000521899
54. Zhang NN, Li XF, Deng YQ, Zhao H, Huang YJ, Yang G, et al. A thermostable mrna vaccine against covid-19. Cell (2020) 182(5):1271. doi: 10.1016/j.cell.2020.07.024
55. Zou C, Xue X, Qian J. Characteristics and comparison of adverse events of coronavirus disease 2019 vaccines reported to the united states vaccine adverse event reporting system between 14 December 2020 and 8 October 2021. Front Med (2022) 9:826327. doi: 10.3389/fmed.2022.826327
56. Zhou Z, Zhu Y, Chu M. Role of covid-19 vaccines in sars-Cov-2 variants. Front Immunol (2022) 13:898192. doi: 10.3389/fimmu.2022.898192
57. Amanpour S. The rapid development and early success of covid 19 vaccines have raised hopes for accelerating the cancer treatment mechanism. Arch Razi Institute (2021) 76(1):1–6. doi: 10.22092/ari.2021.353761.1612
58. Abrams EM, Singer AG, Greenhawt M, Stukus D, Shaker M. Ten tips for improving your clinical practice during the covid-19 pandemic. Curr Opin Pediatr (2021) 33(2):260–7. doi: 10.1097/MOP.0000000000000998
59. Anand P, Stahel VP. Review the safety of covid-19 mrna vaccines: A review. Patient Saf Surg (2021) 15(1):20. doi: 10.1186/s13037-021-00291-9
60. Zheng C, Shao W, Chen X, Zhang B, Wang G, Zhang W. Real-world effectiveness of covid-19 vaccines: A literature review and meta-analysis. Int J Infect diseases: IJID: Off Publ Int Soc Infect Dis (2022) 114:252–60. doi: 10.1016/j.ijid.2021.11.009
61. Ndeupen S, Qin Z, Igyarto BZ. Single-cell suspension preparation from murine organs following in vivo mrna-lnp exposure. STAR Protoc (2022) 3(2):101350. doi: 10.1016/j.xpro.2022.101350
62. Naderi Sohi A, Kiani J, Arefian E, Khosrojerdi A, Fekrirad Z, Ghaemi S, et al. Development of an mrna-lnp vaccine against sars-Cov-2: Evaluation of immune response in mouse and rhesus macaque. Vaccines (2021) 9(9):1007. doi: 10.3390/vaccines9091007
63. Tenforde MW, Self WH, Gaglani M, Ginde AA, Douin DJ, Talbot HK, et al. Effectiveness of mrna vaccination in preventing covid-19-Associated invasive mechanical ventilation and death - united states, march 2021-January 2022. MMWR Morbid mortal weekly Rep (2022) 71(12):459–65. doi: 10.15585/mmwr.mm7112e1
64. Woldemeskel BA, Dykema AG, Garliss CC, Cherfils S, Smith KN, Blankson JN. Cd4+ T cells from covid-19 mrna vaccine recipients recognize a conserved epitope present in diverse coronaviruses. J Clin Invest (2022) 132(5):e156083. doi: 10.1172/JCI156083
65. Ripoll M, Bernard MC, Vaure C, Bazin E, Commandeur S, Perkov V, et al. An imidazole modified lipid confers enhanced mrna-lnp stability and strong immunization properties in mice and non-human primates. Biomaterials (2022) 286:121570. doi: 10.1016/j.biomaterials.2022.121570
66. Sayers EJ, Peel SE, Schantz A, England RM, Beano M, Bates SM, et al. Endocytic profiling of cancer cell models reveals critical factors influencing lnp-mediated mrna delivery and protein expression. Mol therapy: J Am Soc Gene Ther (2019) 27(11):1950–62. doi: 10.1016/j.ymthe.2019.07.018
67. Fedorowski JJ. Could amantadine interfere with covid-19 vaccines based on the lnp-mrna platform? Arch Med science: AMS (2021) 17(3):827–8. doi: 10.5114/aoms/134716
68. Bascomb NF, Turner KJ, Schmidt RR. Specific polysome immunoadsorption to purify an ammonium-inducible glutamate dehydrogenase mrna from chlorella sorokiniana and synthesis of full length double-stranded cdna from the purified mrna. Plant Physiol (1986) 81(2):527–32. doi: 10.1104/pp.81.2.527
69. Ogawa K, Kato N, Yoshida M, Hiu T, Matsuo T, Mizukami S, et al. Focused Ultrasound/Microbubbles-assisted bbb opening enhances lnp-mediated mrna delivery to brain. J Control Release (2022) 348:34–41. doi: 10.1016/j.jconrel.2022.05.042
70. Ryals RC, Patel S, Acosta C, McKinney M, Pennesi ME, Sahay G. The effects of pegylation on lnp based mrna delivery to the eye. PloS One (2020) 15(10):e0241006. doi: 10.1371/journal.pone.0241006
71. Nagy A, Alhatlani B. An overview of current covid-19 vaccine platforms. Comput Struct Biotechnol J (2021) 19:2508–17. doi: 10.1016/j.csbj.2021.04.061
72. Senthilvelan A, Vonderfecht T, Shanmugasundaram M, Pal I, Potter J, Kore AR. Trinucleotide cap analogue bearing a locked nucleic acid moiety: Synthesis, mrna modification, and translation for therapeutic applications. Org Lett (2021) 23(11):4133–6. doi: 10.1021/acs.orglett.1c01037
73. Moore KA, Ostrowsky JT, Kraigsley AM, Mehr AJ, Bresee JS, Friede MH, et al. A research and development (R&D) roadmap for influenza vaccines: Looking toward the future. Vaccine (2021) 39(45):6573–84. doi: 10.1016/j.vaccine.2021.08.010
74. Hein S, Herrlein ML, Mhedhbi I, Bender D, Haberger V, Benz N, et al. Analysis of Bnt162b2- and cvncov-elicited sera and of convalescent sera toward sars-Cov-2 viruses. Allergy (2022) 77(7):2080–9. doi: 10.1111/all.15189
75. Wilson B, Geetha KM. Lipid nanoparticles in the development of mrna vaccines for covid-19. J Drug Delivery Sci Technol (2022) 74:103553. doi: 10.1016/j.jddst.2022.103553
76. Ramachandran S, Satapathy SR, Dutta T. Delivery strategies for mrna vaccines. Pharm Med (2022) 36(1):11–20. doi: 10.1007/s40290-021-00417-5
77. Zhu Y, Yue M, Guo T, Li F, Li Z, Yang D, et al. Pei-Peg-Coated mesoporous silica nanoparticles enhance the antitumor activity of tanshinone iia and serve as a gene transfer vector. Evid Based Complement Alternat Med (2021) 2021:6756763. doi: 10.1155/2021/6756763
78. Ly HH, Daniel S, Soriano SKV, Kis Z, Blakney AK. Optimization of lipid nanoparticles for sarna expression and cellular activation using a design-of-Experiment approach. Mol Pharm (2022) 19(6):1892–905. doi: 10.1021/acs.molpharmaceut.2c00032
79. Li M, Li Y, Li S, Jia L, Wang H, Li M, et al. The nano delivery systems and applications of mrna. Eur J Med Chem (2022) 227:113910. doi: 10.1016/j.ejmech.2021.113910
80. Khurana A, Allawadhi P, Khurana I, Allwadhi S, Weiskirchen R, Banothu AK, et al. Role of nanotechnology behind the success of mrna vaccines for covid-19. Nano Today (2021) 38:101142. doi: 10.1016/j.nantod.2021.101142
81. Vemana HP, Saraswat A, Bhutkar S, Patel K, Dukhande VV. A novel gene therapy for neurodegenerative lafora disease Via Epm2a-loaded dlindma lipoplexes. Nanomed (Lond) (2021) 16(13):1081–95. doi: 10.2217/nnm-2020-0477
82. Ferraresso F, Strilchuk AW, Juang LJ, Poole LG, Luyendyk JP, Kastrup CJ. Comparison of dlin-Mc3-Dma and alc-0315 for sirna delivery to hepatocytes and hepatic stellate cells. Mol Pharm (2022) 19(7):2175–2182. doi: 10.1021/acs.molpharmaceut.2c00033
83. Granados-Riveron JT, Aquino-Jarquin G. Engineering of the current nucleoside-modified mrna-lnp vaccines against sars-Cov-2. BioMed Pharmacother (2021) 142:111953. doi: 10.1016/j.biopha.2021.111953
84. Lasagna A, Lenti MV, Cassaniti I, Sacchi P. Development of hepatitis triggered by sars-Cov-2 vaccination in patient with cancer during immunotherapy: A case report. Immunotherapy (2022) 14(12):915–25. doi: 10.2217/imt-2021-0342
85. Grau S, Martin-Garcia E, Ferrandez O, Martin R, Tejedor-Vaquero S, Gimeno R, et al. Covid-19 mrna vaccines preserve immunogenicity after re-freezing. Vaccines (2022) 10(4):594. doi: 10.3390/vaccines10040594
86. Giannoccaro MP, Vacchiano V, Leone M, Camilli F, Zenesini C, Panzera I, et al. Difference in safety and humoral response to mrna sars-Cov-2 vaccines in patients with autoimmune neurological disorders: The ancovax study. J Neurol (2022) 269(8):4000–12. doi: 10.1007/s00415-022-11142-7
87. Alessa MY, Aledili FJ, Alnasser AA, Aldharman SS, Al Dehailan AM, Abuseer HO, et al. The side effects of covid-19 vaccines and its association with abo blood type among the general surgeons in Saudi Arabia. Cureus (2022) 14(3):e23628. doi: 10.7759/cureus.23628
88. Hajjo R, Sabbah DA, Tropsha A. Analyzing the systems biology effects of covid-19 mrna vaccines to assess their safety and putative side effects. Pathogens (2022) 11(7):743. doi: 10.3390/pathogens11070743
89. Brunello A, Guarneri V, Coppola M, Bernardi M, Ottolitri K, Ghi MG, et al. Safety of covid-19 vaccine in patients with cancer in a high-volume comprehensive cancer center. Oncologist (2022) 27(2):e203–e5. doi: 10.1093/oncolo/oyab037
90. Tarab-Ravski D, Stotsky-Oterin L, Peer D. Delivery strategies of rna therapeutics to leukocytes. J Control Release (2022) 342:362–71. doi: 10.1016/j.jconrel.2022.01.016
91. Gutkin A, Rosenblum D, Peer D. Rna delivery with a human virus-like particle. Nat Biotechnol (2021) 39(12):1514–5. doi: 10.1038/s41587-021-01124-x
92. Zhang Y, Sun C, Wang C, Jankovic KE, Dong Y. Lipids and lipid derivatives for rna delivery. Chem Rev (2021) 121(20):12181–277. doi: 10.1021/acs.chemrev.1c00244
93. Tenchov R, Bird R, Curtze AE, Zhou Q. Lipid nanoparticles-from liposomes to mrna vaccine delivery, a landscape of research diversity and advancement. ACS nano (2021) published online ahead of print, 2021 Jun 28. doi: 10.1021/acsnano.1c04996
94. Rahman MM, Zhou N, Huang J. An overview on the development of mrna-based vaccines and their formulation strategies for improved antigen expression in vivo. Vaccines (2021) 9(3):244. doi: 10.3390/vaccines9030244
95. Qiu M, Li Y, Bloomer H, Xu Q. Developing biodegradable lipid nanoparticles for intracellular mrna delivery and genome editing. Accounts Chem Res (2021) 54(21):4001–11. doi: 10.1021/acs.accounts.1c00500
96. Alasandro M, Choudhury D, Huynh-Ba K, Kochling J, Latoz C, Larkin L, et al. Meeting report: Vaccine stability considerations to enable rapid development and deployment. AAPS Open (2021) 7(1):6. doi: 10.1186/s41120-021-00042-1
97. Dargahi N, Johnson J, Apostolopoulos V. Streptococcus thermophilus alters the expression of genes associated with innate and adaptive immunity in human peripheral blood mononuclear cells. PloS One (2020) 15(2):e0228531. doi: 10.1371/journal.pone.0228531
98. Wang Y, Chen K, Wei Z, Coenen F, Su J, Meng J. Metatx: Deciphering the distribution of mrna-related features in the presence of isoform ambiguity, with applications in epitranscriptome analysis. Bioinformatics (2021) 37(9):1285–91. doi: 10.1093/bioinformatics/btaa938
99. Padda IS, Mahtani AU, Parmar M. Small interfering rna (Sirna) based therapy. Treasure Island (FL: Statpearls (2022).
100. Schoenmaker L, Witzigmann D, Kulkarni JA, Verbeke R, Kersten G, Jiskoot W, et al. Mrna-lipid nanoparticle covid-19 vaccines: Structure and stability. Int J pharmaceut (2021) 601:120586. doi: 10.1016/j.ijpharm.2021.120586
101. Monticolo F, Palomba E, Chiusano ML. Translation machinery reprogramming in programmed cell death in saccharomyces cerevisiae. Cell Death Discovery (2021) 7(1):17. doi: 10.1038/s41420-020-00392-x
102. Shao Y, Xie Z, Liang S, Chen C, Tocher DR, Lin L, et al. Dietary calcium pyruvate could improve growth performance and reduce excessive lipid deposition in juvenile golden pompano (Trachinotus ovatus) fed a high fat diet. Fish Physiol Biochem (2022) 48(3):555–70. doi: 10.1007/s10695-022-01077-1
103. Jung SW, Yeom J, Park JS, Yoo SM. Recent advances in tuning the expression and regulation of genes for constructing microbial cell factories. Biotechnol Adv (2021) 50:107767. doi: 10.1016/j.biotechadv.2021.107767
104. Lane S, Yeomans A, Shakir S. Reports of myocarditis and pericarditis following mrna covid-19 vaccination: A systematic review of spontaneously reported data from the uk, Europe and the USA and of the scientific literature. BMJ Open (2022) 12(5):e059223. doi: 10.1136/bmjopen-2021-059223
105. Hossain GS, Saini M, Miyake R, Ling H, Chang MW. Genetic biosensor design for natural product biosynthesis in microorganisms. Trends Biotechnol (2020) 38(7):797–810. doi: 10.1016/j.tibtech.2020.03.013
106. He Q, Gao H, Tan D, Zhang H, Wang JZ. Mrna cancer vaccines: Advances, trends and challenges. Acta Pharm Sin B (2022) 12(7):2969–2989. doi: 10.1016/j.apsb.2022.03.011
107. Vymetalova L, Kucirkova T, Knopfova L, Pospisilova V, Kasko T, Lejdarova H, et al. Large-Scale automated hollow-fiber bioreactor expansion of umbilical cord-derived human mesenchymal stromal cells for neurological disorders. Neurochem Res (2020) 45(1):204–14. doi: 10.1007/s11064-019-02925-y
108. Nuno-Gonzalez P, Ruiz-Madrigal B, Bastidas-Ramirez BE, Martinez-Lopez E, Segura JE, Panduro A. Expression of apolipoprotein ai mrna in peripheral white blood cells of patients with alcoholic liver disease. Biochim Biophys Acta (2005) 1740(3):350–6. doi: 10.1016/j.bbadis.2004.11.003
109. Rurik JG, Tombacz I, Yadegari A, Mendez Fernandez PO, Shewale SV, Li L, et al. Car T cells produced in vivo to treat cardiac injury. Science (2022) 375(6576):91–6. doi: 10.1126/science.abm0594
110. Gao TA, Chen YY. T Cells to fix a broken heart. Science (2022) 375(6576):23–4. doi: 10.1126/science.abn0851
111. van Doremalen N, Fischer RJ, Schulz JE, Holbrook MG, Smith BJ, Lovaglio J, et al. Immunogenicity of low-dose prime-boost vaccination of mrna vaccine Cv07050101 in non-human primates. Viruses (2021) 13(8):1645. doi: 10.3390/v13081645
112. Martinez-Lopez E, Nuno-Gonzalez P, Ruiz-Madrigal B, Rodriguez-Sancho LC, Hernandez-Nazara ZH, Segura-Ortega JE, et al. Apolipoprotein ai and apolipoprotein e mrna expression in peripheral white blood cells from patients with orthotopic liver transplantation. Liver Int (2007) 27(7):930–7. doi: 10.1111/j.1478-3231.2007.01509.x
Keywords: mRNA, drug, targeted therapy, development, preclinical studies
Citation: Duan Q, Hu T, Zhu Q, Jin X, Chi F and Chen X (2022) How far are the new wave of mRNA drugs from us? mRNA product current perspective and future development. Front. Immunol. 13:974433. doi: 10.3389/fimmu.2022.974433
Received: 21 June 2022; Accepted: 24 August 2022;
Published: 12 September 2022.
Edited by:
Fabio Bagnoli, GlaxoSmithKline, ItalyReviewed by:
Shakti Singh, Lundquist Institute for Biomedical Innovation, United StatesPrashant Kumar, University of Kansas, United States
Copyright © 2022 Duan, Hu, Zhu, Jin, Chi and Chen. This is an open-access article distributed under the terms of the Creative Commons Attribution License (CC BY). The use, distribution or reproduction in other forums is permitted, provided the original author(s) and the copyright owner(s) are credited and that the original publication in this journal is cited, in accordance with accepted academic practice. No use, distribution or reproduction is permitted which does not comply with these terms.
*Correspondence: Xiaodong Chen, chenxd@sj-hospital.org